直接メタノール燃料電池用途向けのさまざまな炭素担体および導電性ポリマー上の白金ベースの触媒:レビュー
要約
白金(Pt)ベースのナノ粒子金属はかなりの注目を集めており、直接メタノール燃料電池(DMFC)の最も一般的な触媒です。ただし、Pt触媒の高コスト、遅い速度論的酸化、およびメタノール酸化反応(MOR)中のCO中間体分子の形成は、単一金属Pt触媒に関連する主要な課題です。最近の研究では、Fe、Ni、Co、Rh、Ru、Co、Sn金属などのPt合金、またはPtの触媒性能を高めるための炭素担体材料の使用に焦点が当てられています。近年、MWCNT、CNF、CNT、CNC、CMS、CNT、CB、グラフェンなどの炭素材料の大きな可能性に支えられたPtおよびPt合金触媒は、優れたMORに貢献できる重要な特性により、注目を集めています。およびDMFCのパフォーマンス。このレビューペーパーでは、DMFCでのPtの使用量の削減、安定性の向上、およびPtの電極触媒性能の向上に関連する上記の合金とサポート材料の開発についてまとめています。最後に、形態、電極触媒活性、構造特性、およびその燃料電池性能の観点から、各触媒と担体について説明します。
はじめに
燃料電池技術は世界中で広く注目されています。燃料電池(FC)は、電気化学反応によって化学エネルギーを電気エネルギーに変換する有望な代替発電技術です[1、2]。さらに、燃料電池技術の場合、燃料電池技術の主な焦点は、低コストの生産を生み出すことであり、それにより、燃料電池システムの強力な性能を達成し、耐久性のある材料を発見する。それにもかかわらず、現在の燃料電池技術で発生する一般的な問題は、システムが高い固有コストと低い耐久性を伴うことです[1]。燃料電池としての可能性にもかかわらず、直接メタノール燃料電池(DMFC)には課題と制限があり、研究者はDMFCの効率と性能を改善する方法を研究するようになります。 DMFCに関する多くの問題が特定されており、未解決のままです。たとえば、アノード電極からカソード電極へのメタノール燃料のクロスオーバー[3,4,5]は、反応速度の遅さ、触媒の不安定性、熱および水管理によるパフォーマンスの低下などです。 [6,7,8]。
最近では、DMFC、プロトン交換膜燃料電池(PEMFC)、固体酸化物燃料電池(SOFC)など、人気のある燃料電池技術である燃料電池の研究が盛んに行われています。新しいエネルギー源として、DMFCはモバイルおよび固定アプリケーションに使用できます[9、10]。燃料電池の分野では多くの研究の進歩が達成されています。燃料電池の中でも、DMFCは、高出力密度、燃料処理のしやすさ、充電のしやすさ、環境の悪さなど、多くの利点があるため、近年広く研究されています[11、12、13、14、15、16]。影響[17、18]。ただし、DMFCの商業化に関するいくつかの技術的課題は、メタノールクロスオーバー、低い化学反応速度、触媒中毒など、未解決のままです。しかし、DMFCは依然として多くの研究者から注目を集めており、低温動作(DMFCシステムは373 Kで動作)により最も人気のある燃料電池になっています。 DMFCの高いエネルギー効率と迅速な起動システムの利点により、DMFCテクノロジーは、住宅用電源、モバイルデバイスのバッテリー、および車両燃料としての適用に非常に適しています[19、20、21、22]。さらに、DMFCの概念をさらに研究して、天然ガスやバイオマスなどの代替燃料源を見つけたり、不安定なエネルギー源への依存を最小限に抑えるために農産物を発酵させてエタノールを生産したりすることもできます[14]。
DMFCでは、アノード側にメタノール溶液が供給され、二酸化炭素(CO 2 )に電気酸化されます。 )以下の反応を通じて:
$$ {\ mathrm {CH}} _3 \ mathrm {OH} + {\ mathrm {H}} _ 2 \ to {\ mathrm {CO}} _ 2 + 6 {\ mathrm {H}} ^ {+} + 6 { \ mathrm {e}} ^ {\ hbox {-}} $$(1)陰極側で陽子が存在する間、(空気からの)酸素は水に還元されます:
$$ 3/2 \ {\ mathrm {O}} _ 2 + 6 {\ mathrm {H}} ^ {+} + 6 \ {\ mathrm {e}} ^ {\ hbox {-}} \ to 3 {\ mathrm {H}} _ 2 \ mathrm {O} $$(2)正味の式DMFC反応は次のように要約できます。
$$ {\ mathrm {CH}} _3 \ mathrm {OH} +3/2 {\ mathrm {O}} _ 2 \ to {\ mathrm {CO}} _ 2 + 2 {\ mathrm {H}} _ 2 \ mathrm { O} $$(3)DMFCシステムには、アクティブモードとパッシブモードの2種類のDMFCモードがあります[23、24、25]。アクティブなDMFCシステムでは、DMFCスタックの出口ストリームは、液体メタノール供給の閉ループ制御を介して再循環されます。一方、アノードストリームの液体メタノールは、目標濃度に基づいてこの燃料を復元するために追加のメタノールと水の十分な注入を提供する上で重要な役割を果たすメタノール濃度センサーによって制御されます。 DMFCシステムでメタノール供給濃度を制御および維持するために使用されるメタノール濃度センサーにはいくつかの種類があります[17]。通常、液体メタノールは蠕動ポンプによってアノード側に送られ、酸素を含む周囲の空気はブロワーまたはファンによってカソード側に供給されます[16]。 DMFCシステムのパッシブモードでは、液体メタノールがシステムに連続的に供給されます。このパッシブコンセプトは、DMFCシステムにとって非常に魅力的です[26、27、28]。パッシブの概念は、システムがサポートデバイスなしで完全に自律的に動作することを意味します。パッシブDMFCの概念は、システムがメタノールをポンプで送り、スタックに空気を吹き込むための外部デバイスの支援なしに完全に自律的に動作することを意味します。 DMFCシステムのパッシブモードでは、触媒層は反応するときにメタノールと酸素によって供給されます。メタノール酸化反応(MOR)中、CO 2 そして、水は受動的な手段、すなわち拡散、自然対流、毛細管現象などによってセルから除去されます[20]。パッシブモードDMFCは、アクティブモードDMFCと比較して、よりシンプルでコンパクトな設計、および低コストの点で有利であるように思われます。複雑なシステム設計と制御は、アクティブモードDMFCの欠点となる可能性があります[21]。実用的な観点から、アクティブモードDMFCは高電力システムでより適切であるように見えますが、パッシブモードDMFCは低電力要件での使用により適しています[22]。
図1は、シングルセルDMFCのセットアップと設計を示しています。シングルセルDMFCスタックは、アノードとカソードの2つのプレートで挟まれた5層の膜電極接合体(MEA)で構成されています。アノード側では、液体メタノール(メタノールと脱イオン水を含む)と無水メタノールが蠕動ポンプによってチャネルに流入します。カソード側では、回転計によって空気が燃料電池に送り込まれます。 DMFCスタックの温度コントローラーは、補助加熱装置によってセル内の動作温度を維持するために使用されます。電子負荷装置は、電流密度をさまざまなレベルに変更し、対応する電圧値を測定するために使用されます。セルの性能は電気化学ワークステーションによって監視され、CO 2 反応全体の最終生成物はCO 2 によって測定されるため 濃度検出器[23]。直接メタノール燃料電池では、実験研究中に考慮しなければならないいくつかの重要な動作パラメータがあります。それは、(i)動作温度、(ii)メタノール濃度、および(iii)供給メタノール溶液と空気の入力流量です[23]。 。図2a、bは、それぞれDMFCのアクティブモードとパッシブモードを示しています。
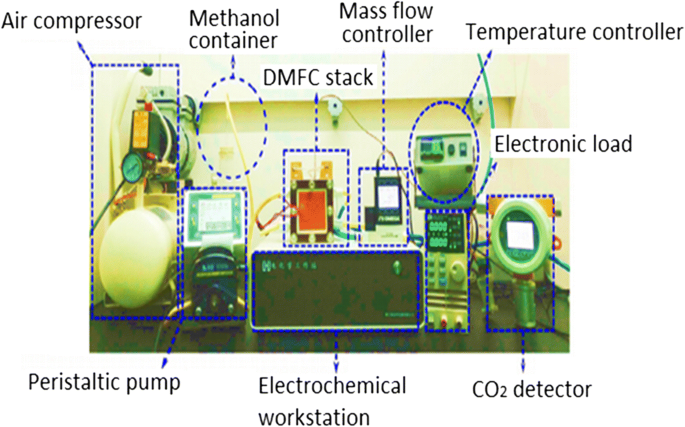
単一セルDMFCの一般的な実験セットアップ[23]
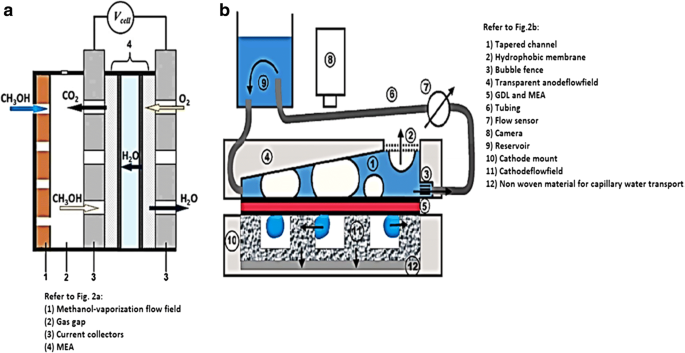
a の概略図 アクティブモード[24]および b DMFCのパッシブモード[25]
このレビューでは、DMFCの貴触媒としてのPt触媒に基づく触媒担体の研究開発における最近の進歩に焦点を当てます。合金、金属、遷移金属、金属炭化物、金属窒化物、およびグラフェン/グラフェンオキシド(G / GO)、カーボンナノチューブ(CNT)、カーボンナノファイバー( CNF)、カーボンナノコイル(CNC)、カーボンブラック(CB)、多層カーボンナノチューブ(MWCNT)、カーボンメソポーラス(CMS)の支持体、および支持体としてのポリアニリン(PANi)やポリピロール(Ppy)などの導電性ポリマー材料。多くの合成方法を適用して、Ptベースの触媒を調製することができます。ナノスケールのPt粒子を得るために適用される最も一般的な方法は、含浸[29,30,31,32,33,34]、熱水技術[35,36,37,38,39,40,41]、マイクロエマルジョン[42,43、 44,45]、および削減[46、47]。一般に、調製方法は触媒粒子の形態とサイズに影響を与える可能性があります。したがって、触媒合成の方法の選択は非常に重要です。
さまざまなタイプのPtベースの触媒の性能
過去10年間、多くの研究者は、DMFCシステム用のメタノールMORでの電極触媒活性を強化するために、電極触媒の開発に研究を集中させてきました[37、38]。白金(Pt)は、MORに対して非常に高い触媒活性を示す単一金属触媒です。ただし、DMFCシステム内の純粋なPtのみは、中間種、つまり一酸化炭素(CO)によって容易に汚染される可能性があり、Pt触媒のコストが高いため、電極触媒としての商業的用途が制限され、それによってメタノール酸化の速度が低下します。 DMFCシステム[48,49,50]。これらの3つのポイントは、DMFCの電極触媒としてPtのみを使用する場合の主な障害と制限です。しかし、これらの障害を克服するために、Ptベースの合金電極触媒を合成して、より少ないPt使用量でより優れた電極触媒性能を達成するためのいくつかの研究が行われています[11、47、51、52]。通常、Pt粒子の平均サイズとその形態は、走査型電子顕微鏡写真(SEM)または透過型電子顕微鏡写真(TEM)分析によって決定できます。これらは、電極触媒の物理的特性を特徴づけるために使用できる触媒分野で最も一般的な方法です。表1は、さまざまな合成方法、特性、およびそれらの性能を使用したPt粒子の平均粒子サイズを示しています。
<図> 図>バイメタルPtRuは、その二官能性メカニズムと配位子効果により、最も活性の高い触媒と見なされています[48、53]。 PtRuは興味深い触媒合金になり、今日まで多くの炭素担体とともに使用されてきました。しかし、ルテニウム(Ru)金属の添加による毒物学的影響は依然として不明です[49]。したがって、「Ptベースの合金の性能」のセクションで説明したように、Ptを他の非貴金属と混合するより安価な合金の研究が行われています[49、50、51、52、54、55、56、57]。
Ptベースの合金の性能
アリコら。 [35]は、MORにおけるPt触媒の触媒活性を高めるために多くの研究が行われていることを発見しました。多くの研究で、最適なPt-Ru比は1:1として特定されており、ナノサイズスケールの粒子サイズは触媒の利用を改善するための理想的なサイズです。ただし、Shi etal。 [38]は、MOR触媒活性を高めるための実験において、3:2がPt-Ruの最適な比率であることを確認しました。それ以外に、PtRu電極触媒粒子が2〜4 nmの範囲のナノサイズの粒子である場合、メタノール電極酸化活性の電極触媒活性も増加する可能性があります。ポーラスら。 [39]この声明に同意した。ご存知のように、Ptはメタノール燃料に対して高い反応性を示し、DMFCシステムのアノード電極の理想的な電極触媒としてPt金属を提供します。それにもかかわらず、Pt触媒のMOR中に、一酸化炭素(CO)、つまり中間種がPt粒子の表面に形成され、触媒表面を汚染します[58,59,60,61]。したがって、Pt粒子表面での有毒種の形成に関連する問題を克服して、Ptの活性部位領域を覆わないようにするために、いくつかの努力が必要です。一般に、PtRu [62,63,64,65,66]、PtRh [67,68,69,70,71]、PtAu [72,73,74]、PtSn [62、63、75、 76,77]、PtNi [64,65,66,67,78,69]、PtCo [70、71、78,79,80]、およびPtFe [81,82,83,84,85]が頻繁に使用されますDMFCシステムのアノード電極用の電極触媒の組み合わせとして。ルテニウム(Ru)、スズ(Sn)、ロジウム(Rh)などのこれらの金属を添加すると、より高い触媒活性が得られると考えられています。
白金ベースの触媒にニッケル(Ni)を組み込むと、MORおよびDMFCに優れた性能が得られます。最新の研究では、Guerrero-Ortegaと共同研究者は、Pt-VulcanサポートにNiを追加すると、バイメタル触媒でのPtの使用量が少なくても、MOR中のファラデー電流の重要な増加が1桁促進されると説明しています。 55]。彼らの実験結果はまた、Niの添加が電極界面でのより良い反応性能を高めるいくつかの構造的および電子的修飾を促進することを示唆しました。別の研究では、AuをPt合金に組み込むと、電子構造が変化し、電気化学的に活性な領域(ECSA)が改善されるため、電極触媒活性が向上しました[47]。一方、Ptベースの合金へのスズ(Sn)の添加は、電気触媒活性の増加を示しました。これは、合金システムへのSnの取り込みと酸化形態の影響を強く受け、酸化電位が低いため、反応を促進します。 56]。また、Ptベースの合金にコバルト(Co)を添加すると、Pt / rGOの10倍のPtCo(1:9)/ rGO触媒によって触媒特性が大幅に向上しました[51]。電流密度の増加は、水の活性化を促進し、CO ads を酸化する、rGOサポートの親水性に対するPtCoナノ粒子の分散の増加に起因します。 Ptサイトで。さらに、Coの二機能メカニズムによれば、H 2 を促進します。 より多くの-OHイオンと他のO 2 を生成するO活性化 PtサイトのCO-中間体種を酸化するための含有種[57]。 Coのこの二官能性メカニズムは、MORへの他の触媒遷移金属にも使用できます。 CO種のCO 2 への触媒酸化メカニズム PtCo触媒の存在下では、次のように要約できます。
$$ \ mathrm {Pt} + {\ mathrm {CH}} _3 \ mathrm {OH} \ to \ mathrm {Pt} \ hbox {-} {\ mathrm {CO}} _ {\ mathrm {ads}} + 4 {\ mathrm {H}} ^ {+} + 4 {\ mathrm {e}} ^ {\ hbox {-}} $$(4)$$ \ mathrm {Co} + {\ mathrm {H}} _ 2 \ mathrm {O} \ to \ mathrm {Co} {\ left(\ mathrm {OH} \ right)} _ {\ mathrm {ads}} + {\ mathrm {H}} ^ {+} + {\ mathrm {e }} ^ {\ hbox {-}} $$(5)$$ {\ mathrm {PtCO}} _ {\ mathrm {ads}} + \ mathrm {Co} {\ left(\ mathrm {OH} \ right) } _ {\ mathrm {ads}} / {\ mathrm {CO}} _ 2 + \ mathrm {Pt} + \ mathrm {Co} + {\ mathrm {H}} ^ {+} + {\ mathrm {e}} ^ {\ hbox {-}} $$(6)さらに、Löffleretal。 [86]は、DMFCのアノード触媒としてPtRuの合成に成功しました。これにより、約50 at。%Ruでメタノール電解酸化用の最も活性な電極触媒が生成されました。一方、Dinh etal。 PtRuが1:1の比率のPtRuは、より強い金属挙動とメタノール酸化(MOR)に対するより高い電極触媒活性を持っていると報告されています[87]。性能はこれらの2つの主要な要因に関連しています:(i)最大化された触媒表面積と(ii)原子比が1:1に近い金属合金サイトの最大数を持つ触媒表面。これらのグループの両方も非常に示した。二機能メカニズムに基づいて、Aricò等。 [58]およびGoodenoughetal。 [62]は、Pt界面活性部位に形成されたCO中間種が二酸化炭素(CO 2 )に酸化される可能性があることを示唆しました。 )低電位領域の二次元素、たとえばRu、Sn、Moに形成された活性酸素原子によって。表1は、MORの研究者によって実施されたさまざまなタイプのPt合金触媒の性能をまとめたものです。二官能性メカニズム[88,89,90]によれば、担持されたPtRu合金触媒のMORは次の式として要約できます。 Ptは、Ruよりもメタノール吸着に対してより活性な触媒です。したがって、メタノール酸化反応のためのPtRu電極触媒の全体的な反応は、二官能性メカニズムに従います。
$$ \ mathrm {Pt} + {\ mathrm {CH}} _3 \ mathrm {OH} \ to \ mathrm {Pt} \ hbox {-} {\ mathrm {CH}} _3 \ mathrm {OH} \ mathrm {ads } \ to \ mathrm {Pt} \ hbox {-} {\ mathrm {CO} \ mathrm {H}} _ {\ mathrm {ads}} \ to 3 \ mathrm {H} +3 \ mathrm {e} \ hbox {-} \ to \ mathrm {Pt} \ hbox {-} {\ mathrm {CO}} _ {\ mathrm {ads}} + {\ mathrm {H}} ^ {+} + {\ mathrm {e}} ^ {\ hbox {-}} $$(7)$$ \ mathrm {Ru} + {\ mathrm {H}} _ 2 \ mathrm {O} \ to \ mathrm {Ru} \ hbox {-} {\ mathrm { OH}} _ {\ mathrm {ads}} + {\ mathrm {H}} ^ {+} + {\ mathrm {e}} ^ {\ hbox {-}} $$(8)$$ \ mathrm {Pt } \ hbox {-} {\ mathrm {CO} \ mathrm {H}} _ {\ mathrm {ads}} + \ mathrm {Ru} \ hbox {-} {\ mathrm {OH}} _ {\ mathrm {ads }} \ to \ mathrm {Pt} + \ mathrm {Ru} + {\ mathrm {CO}} _ {2+} 2 {\ mathrm {H}} ^ {+} + 2 {\ mathrm {e}} ^ {\ hbox {-}} $$(9)$$ \ mathrm {Pt} \ hbox {-} {\ mathrm {CO}} _ {\ mathrm {ads}} + \ mathrm {Ru} \ hbox {-} {\ mathrm {OH}} _ {\ mathrm {ads}} \ to \ mathrm {Pt} + \ mathrm {Ru} + {\ mathrm {CO}} _ 2 + {\ mathrm {H}} ^ {+} + { \ mathrm {e}} ^ {\ hbox {-}} $$(10)この二機能性メカニズムを参照すると、メタノールは最初に解離してPtに吸着され、その後CO ads に分解されます。 および/またはホルミル様種-CHO ads 脱水素反応による(7)。同時に、水はOH ads に解離します Ruサイトに吸着されます(8)。次に、種はPtおよびRuサイトに吸着し、結合してCO 2 を形成します。 分子(9)および(10)。 Pt–CO ads 間の反応 およびRu– OHads CO 2 につながります 進化し、リフレッシュされたPtおよびRuサイトを生成します(反応10)。一方、EwelinaUrbanczykらによって行われた別の作業。 [48]アルカリ性媒体(1.0 M KOH)中でPtNi触媒のメタノール酸化反応を行った。理論的には、アルカリ性媒体でのメタノール酸化反応は次のとおりです。
$$ {\ mathrm {CH}} _3 \ mathrm {OH} +6 \ mathrm {OH} \ to {\ mathrm {CO}} _ 2 + 5 {\ mathrm {H}} _ 2 \ mathrm {O} +6 { \ mathrm {e}} ^ {\ hbox {-}} $$反応はDMFCのPt電極で二酸化炭素に始まります。このプロセス中に、中間分子(CO)が形成され、Pt活性側の毒と不活性化を引き起こす可能性があります。このCO分子は、メタノールの不完全な酸化の産物です。不完全なメタノール酸化は、中間生成物としてCOを形成します(式11)。電極触媒表面は、ヒドロキシル基を吸着することもできます(式6)。最後に、主生成物の脱着により、二酸化炭素が生成されます(13)。メタノールの酸化中に生成される可能性のある2番目の毒はメタンです。この場合、次のような反応が起こる可能性があります(8)。電気化学反応における中間形態の炭素の二酸化炭素への全酸化は次のとおりです。
$$ 3 \ mathrm {Pt} + {\ mathrm {CH}} _3 \ mathrm {OH} \ to \ mathrm {Pt}-\ mathrm {COads} +4 {H} ^ {+} + 2 \ mathrm {Pt } + 4e- + {H} ^ 2O $$(11)$$ \ mathrm {Ni} + {H} _2O \ to \ mathrm {Ni}-\ mathrm {OHads} + {H} ^ {+} + e -$$(12)$$ \ mathrm {Pt}-{\ mathrm {CO}} _ {\ mathrm {ads}} + \ mathrm {Ni}-\ mathrm {OHads} \ to {\ mathrm {CO}} _2 + {H} ^ {+} + \ mathrm {Pt} + \ mathrm {Ni} + e- $$(13)$$ \ mathrm {Pt}-{\ mathrm {CH}} _3 + \ mathrm {Pt}- H \ to 2 \ \ mathrm {Pt} + {\ mathrm {CH}} _ 4 $$(14)$$ \ mathrm {Pt}-{\ mathrm {CH}} _3 + \ mathrm {Ni} {\ left(\ mathrm {OH} \ right)} _ 2 \ to \ mathrm {Pt} + {\ mathrm {CO}} _ 2 + \ mathrm {Ni} +5 {H} ^ {+} + 5e- $$(15)現在、研究者は、PtRuSn [91、92]、PtRuNi [93,94,95]、PtRuMo [70、96 、97]、第四級PtRuOsIr [79、80]、およびPtRuIrSn [97、98]は、MORでの優れた挙動と、Pt表面サイトに形成される中間種(CO)の除去のためです。しかし、これらの三元および四元触媒への第3および第4の金属の添加はまだ不明です。さらに、三元および四元合金の製造にはいくつかの制限と課題があります。金属と組成の多くの可能な組み合わせのために、触媒形態と触媒組成の最適化を得るのは困難になります。ただし、多くの研究により、3番目と4番目の金属の添加により、触媒活性が著しく向上し、触媒の安定性が向上し、メタノールの電気酸化とDMFCアプリケーションに対するCO耐性が良好であることが証明されました。
Tsiouvaras etal。 [99]は、PtRuMo / C触媒の電気化学的測定を実施し、すべての三元触媒は二元触媒よりもCOおよびメタノール酸化に対して活性が高いが、触媒はH 2 で処理されることを発見しました。 Heで処理された、または処理されていない三元触媒と比較して、約15%改善された性能を示した。 2012年、Hu etal。 [100]は、優れたバイメタル(PtNi)電極触媒、つまり中空メソポーラスPtNiナノスフェア(HMPNN)の合成に成功しました。この触媒は、HMPNNの独自の構造とその大きな電気化学的表面積により、MORで優れた触媒性能を示し、Ptの利用効率が大幅に向上しました。 2016年頃、Yang etal。 [101]はまた、合成されたバイメタルPtFe電極触媒の反応性を調査し、Ptと鉄(Fe)金属間の強い相互作用がバイメタルNPの吸着エネルギーを低下させる可能性があることを観察しました。彼らはまた、Fe原子と単一空孔グラフェンの間の相互作用がPt原子と単一空孔グラフェン。図3は、Yang et al。によって提案された、グラフェン支持体上に分散したPtおよびFe粒子の位置を示しています。 [101]。
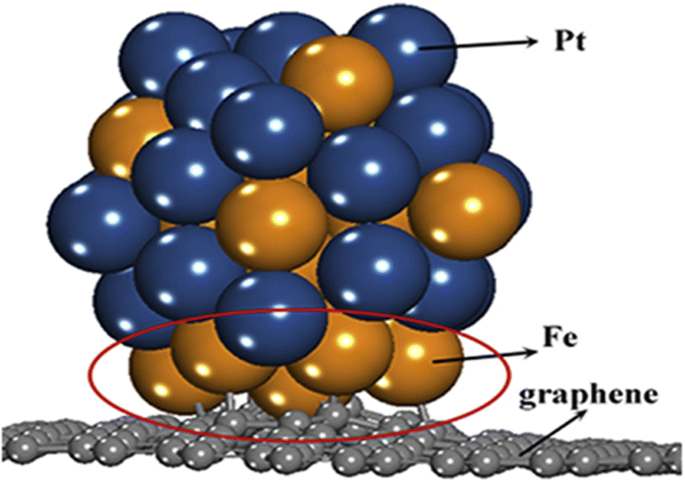
ヤンらによって説明されたグラフェン支持体上のPtFe触媒の位置。 [101]
Ptベースの触媒と遷移金属炭化物の性能
腐食に対する高い機械的および化学的安定性、酸性環境に対する優れた耐性、長期安定性、および高いCO耐性を備えた遷移金属炭化物(TMC)は、アノード触媒として機能することができます[88、89、90、102、103、104]。さらに、TMCは、活性、選択性、および耐毒性に関して、親金属と比較して多くの利点も提供します。たとえば、炭化タングステン(WC)は、優れた導電性、酸性環境への耐性、低コストなどの特殊な特性を示します。 、およびメタノール電解酸化プロセスにおけるCO中毒に対する耐性[88、105、106]。
王ら。 [103]は、高表面積(256 m 2 )の合成を報告しました g -1 )単純な水熱法による炭化タングステンミクロスフェア。 W 2 合成されたままのサンプルでは、Cが主相として検出されました。現在、研究者は現在、WCに担持されたPtがDMFCの理想的な触媒としての可能性を模索しています[38、88、107、108]。クリスチャン等。 [106]は、遷移金属元素と比較して、TMCは、水素、一酸化炭素、アルコールの酸化反応や酸素の還元など、特定の化学的および電気化学的反応に対して、Pt、Pd、Rh、Ruなどの貴金属のように振る舞うと結論付けました。 [109、110]。別の研究では、劉等。 [107]は、炭化モリブデン(Mo-Carbides)が炭化タングステンの促進剤として作用し、DMFCの電極触媒活性を高める可能性があることを示しました。ただし、Pt金属を組み込まない場合、DMFCシステムのMORに対する純粋なWCの電極触媒活性は依然として低くなります。したがって、WCコンポーネントに少量の白金金属を添加すると、PtとWCの相乗効果を利用するのに非常に便利です[91、111、112]。一方、Hassan etal。 [109]は、メタノールの酸化中に形成される一般的な不純物(CO種)がPt表面に強い結合エネルギーを持っていることを明らかにしました。したがって、Pt活性部位から除去できるように酸化する必要があります。 Pt / WC電極触媒へのWC成分の添加は、MORに対して高いCO耐性を示しており、これは、支持成分としてのPt金属とWCとの間に相乗効果が存在することを示している。この研究は、優れた電極触媒性能を維持しながら、Ptのコストを削減するために、より少ないPt金属を使用することによって別の研究者によっても実行されました。
それ以外に、WC成分はメトキシ基(CH 3 )の形成に対してより活性があります。 O-)純粋なPtよりも[113、114]。 (Pt:Ru)4-WC / RGOのCV性能は、330.11 mA mg -1 の電流密度で卓越した触媒性能を示します。 他の5つの触媒と比較したPtは、合成されたままの電極触媒がMORに対して優れた触媒活性を持っていることを示しています。さらに、Pt触媒上でのRuとWCの組み合わせにより、OH表面量が増加し、Pt表面に吸着されたCOがより低い電位で酸化されるようになりました[39]。
Ptベースの触媒と遷移金属窒化物の性能
遷移金属窒化物(TMN)は、その優れた導電性(金属)、硬度、高い電気化学的安定性、および燃料電池の動作条件下での耐食性により、Pt触媒担体として理想的な候補です[115、116、117、118]。 CrsN、TiN、VNなどの遷移金属窒化物に担持されたPt触媒が報告されており、従来の炭素担体と比較して高い触媒性能と優れた安定性を示しました[112]。第8族、第9族、および第10族の金属(Ru、Os、Rh、Ir、Pd、およびPt)の2列目と3列目を除いて、すべての遷移金属が窒化物を形成できます。遷移金属窒化物の挙動と構造特性は、文献[92,93,94]に記載されています。 Xiao etal。 [112]は、酸素還元反応(ORR)に対して優れた性能と安定性を示した窒化チタンコバルト担持Pt電極触媒を調製しました。 Ti 0.9 Co 0.1 N担持Pt触媒は、小さな粒子サイズと良好な金属分散を示しました。この調製された電極触媒はまた、Ptの電気化学的表面積(ECSA)を維持し、ECSAの保存を大幅に改善し、10,000 ADTサイクル後の初期ECSA低下はわずか35%減少しました。コバルトドーピングは、ORR活性と耐久性を大幅に向上させました。一方、DMFCシステムの高性能で耐久性のある電極触媒は、大きな表面積のPt(Ru)/ TiN電極触媒を使用して得ることができます。これは、MORに対して高い電気化学的活性を示し、触媒活性が約52%向上し、安定性/耐久性が良好です。商用JM-Pt(Ru)へ。一方、DMFCの単一セル性能は、最大電力密度を56%向上させ、CSG-Pt(Ru)/ TiN電極触媒の優れた電気化学的安定性を実証しました[115]。
中空で多孔質の構造と高表面積を備えた窒化チタン鉄ナノチューブに担持されたPtナノ粒子に関する現在の研究は、Liらによって合成されました。 [116]。それは、酸性条件でのMORに対する電極触媒活性の有意な増加を示し、より優れた耐久性を示した。これらの特性の理由は、Feの添加がPt原子の電子構造を調整できることを検証した実験データによるものであり、これはMOR用のPt触媒の強化された活性と安定性に貢献します。一方、シャオらによって行われた以前の仕事では。 [117]、Pt / Ti 0.8 Mo 0.2 N触媒は、多孔質構造と高表面積、小型で十分に分散したPtナノ粒子を示しました。この触媒システムは、TiNナノ構造の固有の電気化学的安定性を維持し、MOR活性と耐久性を著しく向上させました。ただし、窒化タングステン(WN)の電気化学的安定性に関する現在入手可能な情報はまだ不十分です[109]。
一方、MoxN( x =1または2)Ti基板上で4.4 M H 2 の酸性電解質中で電気化学的安定性を示した SO 4 50回の繰り返しサイクルで最大+0.67 V(対SHE)の陽極電位[110]。ただし、この電極触媒は、高陰極(-0.1 V対SHE未満)および陽極(+ 0.67 V対SHE以上)の電位領域で、それぞれ陰極腐食および陽極腐食による亀裂や崩壊などの表面損傷を示しました。 + 0.67 V(vs。SHE)を超える高陽極電位領域では、MoOx酸化物の形成により酸素の組成が増加し、失活を引き起こす可能性があります。これらの結果は、MoxNが水性電解質に存在する酸素種と反応し、+ 0.67 V(vs。SHE)を超えると不安定になることを示しています。 Mustafha etal。 [111]は、支持体としてTiNにロードされたPtがメタノール酸化に対して電気活性を示し、高いIf / Ib比が、0.5 M CH で20mV / sのスキャン速度で実行されたボルタモグラムの高いCO耐性を表すことを発見しました。 3 OH + 0.5 M H 2 SO 4 電解質として。 Pt / TiNのCO抵抗の原因として、PtとTiNとの間の二機能効果が挙げられた。さらに、Ottakam Thotiyl etal。 [91]は、PtをロードしたTiN触媒で良好な結果を達成し、メタノールの電気化学的酸化に対して非常に優れたCO耐性を示しました。彼らは、アルカリ性媒体中のMORのPtサポートとして適したTiNの特別な特性は、並外れた安定性、極端な耐食性、優れた電子伝導性、および強力な接着挙動を示すことであると結論付けました。 TiN-supported catalysts are beneficial in terms of long-term stability, exchange current density, and stable currents at low overpotential. Platinum loadings of 40 wt% on TiN were used in the experiments.
In recent years, Liu et al. [118] successfully synthesized platinum on titanium nickel nitride decorated 3D carbon nanotubes which reduced graphene oxide (TiNiN/CNT-rGO) support by solvothermal process followed by nitriding process. Pt with small particle size is well-dispersed on TiNiN/CNT-rGO support. The 3D shape of CNT-rGO support gives a fast route for charge transfer and mass transfer as well as TiNiN NPs with good synergistic effect and the strong electronic coupling between different domains in TiNiN/CNT-rGO support. Thus, it greatly improved the catalytic activity of this catalyst. In another research, the non-carbon TiN nanotubes-supported Pt catalyst done by Xiao et al. [119] also displayed enhanced catalytic activity and durability toward MOR compared with the commercial Pt/C (E-TEK) catalyst.
Performance of Pt-Based Catalysts with Transition Metal Oxide
パンら。 [92] reported the synthesis of platinum–antimony-doped tin oxide nanoparticles supported on carbon black (CB) as anode catalysts in DMFC, which exhibited better improvement in catalytic activity toward MOR compared to Pt-SnO2 /C or commercial Pt/C electrocatalyst. The enhancement in activity was attributed to the high electrical conductivity of Sb-doped SnO2 , which induced electronic effects with the Pt catalysts. Another work done by Abida et al. [93]described the preparation of Pt/TiO2 nanotube catalysts for methanol electrooxidation. TiO 2 nanotubes-supported Pt catalyst (Pt/TiO2 nanotubes) exhibited excellent catalytic activity toward MOR and had good CO tolerance. They also reported that the use of hydrogenotitanate nanotubes as a substrate for the Pt catalyst considerably improved the COads oxidation on Pt, but the MOR still occurred at high potential. Then, several years later, Wu et al. [94] synthesized Pt-C/TiO2 with MOR activity 1.6 higher than commercial Pt-C and the stability of Pt-C/TiO2 was also enhanced by 6.7 times compared to Pt-C. The excellent performance of this catalyst was a contribution of mesopores and partially coated carbon support. Zhou et al. [95] prepared hollow mesoporous tungsten trioxide microspheres (HMTTS) using the spray-drying method to yield Pt/HMTTS. The electrocatalyst exhibited excellent electrocatalytic activity and high stability toward MOR than Pt/C and Pt/WO3 electrocatalysts, which may be attributed to the well-ordered Pt particles (with an average size of 5 nm) on the HMTTS surface.ウーら[120] used polystyrene spheres as templates to obtain pore-arrayed WO3 (p-WO3 )。 The Pt nanoparticles with an approximate size of 3.3 nm dispersed on pore-arrayed WO3 (Pt/p-WO3 ) exhibited high catalytic activity toward MOR.
Li etal。 [121] used Sn-doped TiO2 -modified carbon-supported Pt (Pt/Ti0.9 Sn0.1 O 2 –C) as an electrocatalyst for a DMFC system. The synthesized Pt/Ti0.9 Sn0.1 O 2 –C electrocatalyst revealed high catalytic activity and CO tolerance toward MOR. The enhanced catalyst activity was due to the high content of OH groups on the Ti0.9 Sn0.1 O 2 electrocatalyst sample and the strengthened metals and support interactions. In addition, Lv et al. [122] also reported in their work that the addition of TiO2 could not only facilitate CO removal and hinder CO formation on Pt surface during methanol oxidation, but it can also prevent the agglomeration and corrosion of Pt, which can be concluded from strong metal-supports interaction between TiO2 –C and Pt. Huang etal。 [123] revealed that a TiO2 -coated carbon nanotube support for Pt electrocatalysts could be prepared via a one-step synthesis. Hao et al. [124] developed a new catalyst composed of Pt nanoparticles deposited on graphene with MoO3 。 These catalysts exhibited high catalytic activity toward MOR and high resistance to CO species. However, the size of MoO3 must be tuned by controlling the metal oxide loading.
The selection of metal oxide such as MnO, RuO, CeO, SnO2 , MgO, and V2 O 5 as additional component in electrocatalyst of Pt because of their low cost, good electrochemical properties, and have proton-electron intercalation properties [125]. From the catalytic activity aspect, it can be summarized that the addition of these metal oxides can enhance the electrocatalytic activity of DMFC and other fuel cells. The incorporation of these conducting metal oxides together with Pt catalyst could also facilitate the oxidation process of CO intermediate molecules. Hence, these types of metal have high potential to be used together with platinum as anode electrode.
Carbon support
To improve the utilization of the Pt catalysts, the carbon support is also another useful approach to be used together with Pt. Carbon materials are largely used as catalyst support because of its special properties such as relatively stable in both acid and basic electrolyte, good conductivity, and provide high surface area for dispersion of metal catalyst. It is believed that carbon materials have a strong effect that can influence the electrocatalysts properties such as metal particle size, morphology, metal dispersion, alloyed degree, and stability. Carbon supports can also affect the performance of supported catalysts in fuel cells, such as mass transport and catalyst layer electronic conductivity, electrochemical active area, and metal nanoparticle stability during the operation.
Currently, a great concern of the development in the nanotechnology field, especially carbon nanomaterials synthesis, is to create more stable and active supported catalysts. Support materials of nanoparticles are believed to be the most promising materials for catalytic activity in fuel cells, including the DMFC system. Pt has been traditionally used as nobel catalysts for many fuel cells application [126,127,128]. However, the high cost and low reserve are hindering commercialization of fuel cells and driving researchers to make the utmost of the catalyst. According to this problem, the major effort has been done toward nanoscaling of the catalyst nanoparticles to form more active sites per mass unit. The morphology, structure, and activity of the catalyst, and correspondingly the whole lifetime of a cell, thus strongly depend on the catalyst support [129]. Table 2 shows the preparation, physical properties, performance, and activity of Pt-based supported carbon done by groups of researchers. The details of Pt-based supported carbon will be performed in the following sections:“Graphene Support” to “Carbon Nanocoils”.
<図> 図>Graphene support
Graphene has many extraordinary properties; it exists as a two-dimensional carbon (2-D) form, which is called a crystalline allotrope, one-atom-thick planar flat sheet of sp2 tightly bonded carbon atoms with a thickness of 0.34 nm. Its carbon atoms are packed in a regular atomic-scale chicken wire (hexagonal) pattern [92, 119]. The theoretical specific surface area of graphene is 2630 m 2 g -1 , which is much larger than that of carbon black (typically less than 900 m 2 g -1 ) and carbon nanotubes (100 to 1000 m 2 g -1 ) and similar to that of activated carbon [130]. Graphene has high potential as a metal support [131, 132, 133] [33] due to its high surface area [134] for better catalyst/metal dispersion [135], high electrical conductivity [136], and good thermal properties [137, 138]. Moreover, the functionality of graphene support can be modified by changing it surface structure, and hence contribute to its potential applications, such as in fuel cells, energy storage, electrochemistry, supercapacitors, and batteries [138,139,140,141,142]. Figure 4 illustrates the preparation steps to obtain the graphene nanosheets (GNS), while Fig. 5 shows their TEM images [143]. It can be clearly observed that the thickness of the GO with many typical wrinkles obviously decreases compared to graphite. This can be explained by the presence of the rich oxygen-containing functional groups over the surface of GO [132]. Besides, both resulting GN-900 and GN-900-C contained of a large size of nanosheets structure, but the GN-900-C comprised more transparent than the GN-900.
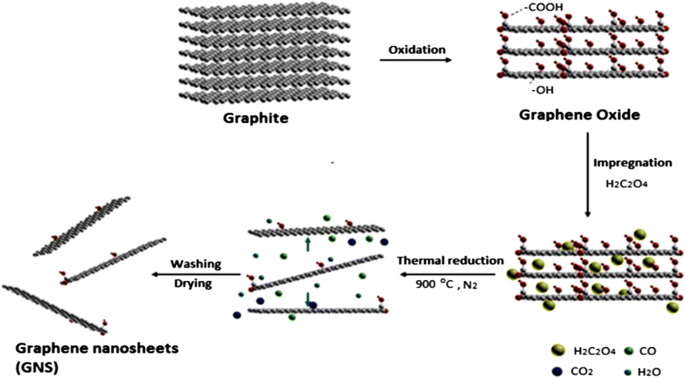
illustration of the preparation of graphite oxide to graphene nanosheets (GNS) by using oxalic acid [143]
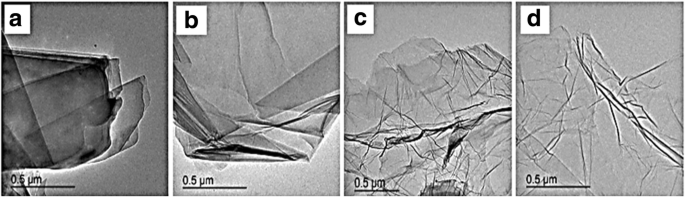
TEM images of graphite (a ), GO (b ), GN-900 (c ), and GN-900-C [143]
The discovery of graphene sheets began around 2000 by mechanical extracting process from 3D graphite source [133]. Graphene can be obtained by several synthesis methods such as hydrothermal [144], chemical reduction [143], chemical vapor deposition, and electrochemical. Ma et al. [145] enhanced the electrocatalytic activity of Pt nanoparticles by supporting the Pt nanoparticles on functionalized graphene for DMFC. Functionalized graphene was prepared by methyl viologen (MV) and Pt/MV–rGO electrocatalyst was synthesized by a facile wet chemical method. They also reported that the higher catalytic activity of Pt/MV–RGO was attributed to the synergetic effect between MV and rGO.
Meanwhile, Zhang et al. [146] modified the graphene support with graphene nanosheets through Hummer’s method, followed by polymerization of aniline (as nitrogen source). The TEM images for Pt/NCL-RGO and Pt/RGO electrocatalysts show that the aggregation between separated graphene sheets was decreased by nitrogen-doped carbon layer (NCL), leading to a better dispersion of the Pt catalyst on the graphene nanosheets support and better electroactivity and stability toward methanol electrooxidation (MOR). Presence of NCL successfully prevented the aggregation of graphene nanosheets as the Pt nanoparticles supporting material.
In 2011, Qiu et al. [135] successfully synthesized nanometer-sized Pt catalyst via sodium borohydride reduction method with an average particle size of only 4.6 nm. These Pt nanoparticles showed an even dispersion of Pt catalyst on graphene oxide support and very high electrocatalytic activity toward MOR by controlling the percent deposition of Pt loaded on the graphene. In another study conducted by Ojani et al. [147], for synthesized Pt-Co/graphene electrocatalyst, it was shown that graphene nanosheets improved the electrocatalytic behavior and long-term stability of the electrode. In addition, the Pt-Co/G/GC electrocatalyst showed great stability toward MOR. The catalytic performance toward MOR can also be improved by using cobalt core–platinum shell nanoparticles supported on surface functionalized graphene [148]. This enhanced catalytic activity could be attributed to the poly (diallyldimethylammonium chloride) (PDDA) that plays a crucial role for dispersion and stabilization of Co@Pt catalyst on graphene support. PDDA-functionalized graphene provided the higher electrochemical active surface area [149, 150]. Huang etal。 [138] also studied a PtCo-graphene electrocatalyst with outstanding catalytic performance and high CO tolerance toward the MOR, which far outperformed Pt-graphene and PtCo-MWCNT electrocatalysts with the same ratio of Pt and carbon content. Figure 4 shows the formation of a graphene-PtCo catalyst prepared from a graphite source. Sharma et al. [57] synthesized Pt/reduced graphene oxide (Pt/RGO) electrocatalyst using a microwave-assisted polyol process, which sped up the reduction of GO and formation of Pt nanocrystals. They compared Pt/RGO to a commercial carbon support (Pt/C), which exhibited high CO tolerance, high electrochemically active surface area, and high electrocatalytic activity for the MOR. In a previous study, Zhao et al. [139] reported that the unique 3D-structured Pt/C/graphene aerogel (Pt/C/GA) exhibited greater stability toward MOR with no decrease in electrocatalytic activity. Moreover, the Pt/C/graphene aerogel also exhibited significantly higher stability to scavenge crossover methanol at high potential in an acidic solution compared with the commercial Pt/C electrocatalyst. At the initial catalytic stage, the Pt/C electrocatalyst lost approximately 40% after 1000 CV cycles. In contrast, the Pt/C/graphene aerogel only lost 16% of the initial catalytic activity. After 200 cycles of CV, the current density of Pt/C/graphene aerogel was much higher with a remarkably higher stability than that of Pt/C electrocatalyst. Meanwhile, Yan et al. [151] demonstrated highly active mesoporous graphene-like nanobowls supported Pt catalyst with high surface area of 1091 m 2 g -1 , high pore volume of 2.7 cm 3 g -1 , and average pore diameter of 9.8 nm obtained by applying a template synthesis method. In addition, the Pt/graphene bowls also achieved high performance toward MOR with a current density value of 2075 mA mgPt -1 , which was 2.87 times higher than that of commercial Pt/C (723 mA mgPt -1 )。 The onset potential for the Pt/graphene bowls toward methanol electrooxidation was negatively shifted by approximately 160 mV compared with that to the latter and showed CO resistance. Figure 6 shows the proposed schematic for the formation of PtCo catalyst on reduced-GO (rGO) support [51]. It is described that the formation of graphene oxide nanosheets from oxidation of graphite powder leads to the increase in interlayer “d” spacing of stacked graphitic sheets from 0.34 to 0.78 nm due to the presence of various oxygen-containing functional groups. The oxygen-containing functional groups act as anchor sites for the well-dispersed Pt and PtCo nanoparticles on rGO sheets, and used for efficient electrooxidation of methanol.
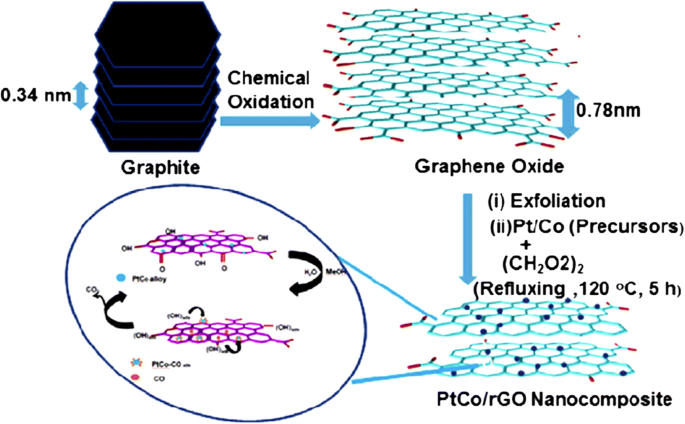
Illustrates the schematic formation of graphene supported Pt-Co catalyst [51]
We can conclude that the reduce graphene oxide (rGO), graphene, modified graphene as supporting material exhibited high electrocatalytic activity toward methanol electrooxidation process. A lot of studies have been reported related to the particle size distribution and size, morphologies, and catalytic activities of Pt and Pt alloys using graphene as supporting material, which showed great improvement in fuel cell performance as mentioned and discussed above. Thus, graphene support can be further studied for better fuel cell performance.
Multiwall Carbon Nanotube and Single-Wall Carbon Nanotube Support
Several years ago, Jha et al. [140] prepared multiwall carbon nanotube (MWCNTs) via chemical vapor deposition using an AB3 alloy hydride catalyst. Platinum-supported MWCNT (Pt/MWCNT) and platinum-ruthenium-supported MWCNT (Pt-Ru/MWCNT) electrocatalysts were prepared by chemical reduction. The performance of these electrodes was studied at different temperatures, and the results demonstrated a very high power density of 39.3 mW cm −2 at a current density of 130 mA cm −2 , which could be attributed to the dispersion and accessibility of the MWCNT support and Pt-Ru in the electrocatalyst mixture for the methanol oxidation reaction. This was also done by other researchers that using different catalyst supported MWCNT for DMFC system [152,153,154,155]. Meanwhile, Wu and Xu [156] compared MWCNT-supported Pt and single-wall carbon nanotube (SWCNT)-supported Pt. Figure 7 shows that the TEM images of Pt catalyst was deposited on MWNT and SWNT electrodes through the electrodeposition technique. The Pt particles in Pt-SWNT (Fig. 7b) looked closer contact with the network of entangled and branched bundles of SWNT support, and the shape is closer to highly exposed sphere. The benefits of the SWCNT support are due to its greater electrochemical surface-active area and easier charge transfer at the electrode/electrolyte interface because of the graphitic crystallinity structure, rich amount of oxygen-containing surface functional groups, and highly mesoporous and unique 3D-structure of SWNT. The electrodeposition technique carried out by them contributed to higher utilization and more uniform dispersion of Pt particles on its support.
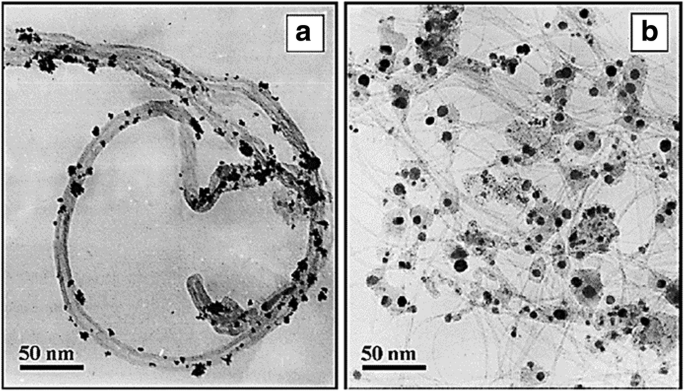
TEM images for the Pt on MWCNT(a ) and SWCNT (b ) [156]
Then, Wang et al. [157] reported the high performance of modified PtAu/MWCNT@TiO2 electrocatalyst prepared via deposition-UV-photoreduction for DMFC, which also exhibited high CO tolerance.趙ら[126] studied 3D flower-like platinum-ruthenium (PtRu) and platinum-ruthenium-nickel (PtRuNi) alloy nanoparticle clusters on MWCNTs prepared via a three-step process, and the best ratios obtained from their experiments for the PtRu and PtRuNi alloys were 8:2 and 8:1:1, respectively. Another group, i.e., Zhao et al. [158], found a higher current density toward MOR and better activity for MWCNT-supported PtWC compared with Pt/C electrocatalyst. These results were attributed to the factors of the synergistic effect between the Pt catalyst and the WC component, high CO tolerance from the bifunctional effect of the Pt catalyst and the WC component, and strong interaction between metals and WC in the electrocatalyst composite.
As a summary, both of MWCNT and SWNT support in terms of structural, surface, and electrochemical properties have their own characteristics as supporting material that remarkably enhanced their performance in catalysis of methanol oxidation process. However, as a comparison, SWCNT possess a high degree of graphitization, highly mesoporous 3D structure, and contain more oxygen-containing functional groups at its surface sites. In relation with these properties, the SWCNT exhibits a higher electrochemically accessible surface area and faster charge transfer rate at the electrode/electrolyte interface.
Carbon Nanotube Support
Wen et al. [144] proposed that carbon nanotubes (CNTs) support could improve fuel cell performance; for example, Pt can be fixed to the inner wall and the outer wall of CNTs and may cause improvement in the electrocatalytic properties of platinum-CNTs. Yoshitake et al. [159] proposed that fuel cells using CNTs as the catalyst support produced larger current densities. The addition of binary or other components to the electrocatalysts for methanol electrooxidation overcomes the problems related to catalyst poisoning caused by CO during the reaction. Therefore, new electrocatalyst carbon supports, such as carbon nanotubes [160, 161], are being actively developed to significantly improve fuel cell performance. Kakati et al. [128] successfully synthesis the PtRu on CNT/SnO2 for anode catalyst DMFC via hydrothermal process. It has been found that the presence of SnO2 provide a high durability property for the catalyst and the presence of SnO2 in the district of Pt could supply oxygen-containing functional groups for the removal of CO intermediate molecules from the Pt surface sites during electrooxidation of methanol. Generally, the decomposition methanol occurs at Pt surface sites; meanwhile, the decomposition of water occurs at SnO2 surface sites to form oxygen-containing species which then react with CO intermediate molecules. However, as support material, the conductivity property of SnO2 still needs to be enhanced. Kakati et al. [128] also proposed the schematic diagram of the formation of PtRu on CNT/SnO2 composite as shows in Fig. 8, and FESEM images of CNT/SnO2 composite support (a and b) and PtRu/SnO2 /CNT composite electrocatalyst (c and d) in Fig. 9.
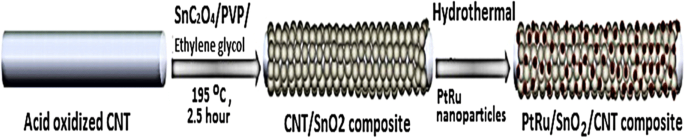
Illustrates the schematic diagram for the formation of PtRu/SnO2/CNT composite [128]
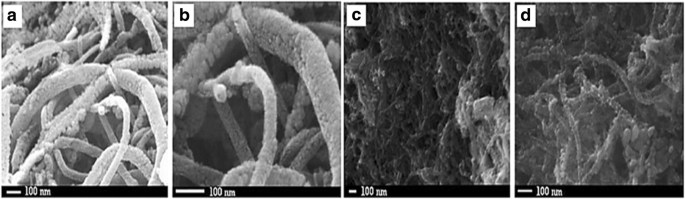
FESEM images of CNT/SnO2 composite support (a 、 b ) and PtRu/SnO2/CNT composite electrocatalyst (c 、 d )
Chien etal。 [127] proposed that the high catalytic performance of Pt-Ru/CNT for MOR can be attributed to the presence of CNT as the carbon support material with several factors:(i) the as-synthesized Pt-Ru/CNT electrocatalyst owns the ideal nanosized particles and composition to increase catalytic activity, (ii) the presence of functional group on the CNT surface results in high hydrophilicity of CNT, which produces better electrochemical reaction on the electrode area, and (iii) the high electronic conductivity of the CNT support lowers the resistance in MOR. Jeng et al. [150] prepared Pt-Ru/CNT electrocatalyst via a modified polyol with a PtRu composition ratio of 1:1, exhibiting high catalytic activity toward MOR and better performance than that of commercial PtRu/C. Show et al. [162] reported that Pt catalyst with a size of less than 10 nm can be obtained by dispersing the Pt particles on a CNT surface using the in-liquid plasma method, and excellent performance was demonstrated by the electrical power achieving 108 mW cm −2 [162]. The in-liquid plasma method was also used by Matsuda et al. [163] that can applied to obtain nanometer-sized Pt catalyst on support material that remarkably enhanced the fuel cell performance.
To be concluded, high electric conductivity, large surface area, excellent chemical and electrochemical stabilities, quasi one-dimensional structure, and good morphology as the supporting materials are the key factors of carbon nanotubes (CNTs) in enhancing the DMFC performance. In addition, carbon support materials such as CNTs which contribute a large effect on metal distribution and size have also been proven to be an essential to the electrocatalysts to achieve high catalytic activity during methanol oxidation process.
Carbon Nanofiber Support
Steigerwalt et al. [164] reported the successful synthesis of PtRu alloy that was widely dispersed on a graphene carbon nanofiber (CNF) support as an electrocatalyst in DMFC. The catalytic activity was enhanced by ~ 50% relative to that recorded for an unsupported PtRu colloid anode electrocatalyst. Meanwhile, Wang et al. [152] reported that Pt/CNF nanocomposites obtained by the reduction of hexachloroplatinic acid (H2 PtCl 6 ) precursor with formic acid (HCOOH) in aqueous solution containing electrospun CNFs at room temperature showed a higher current density than other prepared Pt/CNFs and was approximately 3.5 times greater than that of the E-TEK Pt/C electrocatalyst. Another research carried out by Giorgi et al. [153] described a CNF and bimetallic PtAu electrode with a single layer and both diffusive and catalytic functions using a decreased noble metal amount (approximately five times less) with a consequent large cost reduction. In addition, the bifunctional electrocatalytic properties were also active for the MOR on the PtAu nanoparticle catalysts [154]. Calderón et al. [155] reported PtRu/CNF prepared via reduction using sodium borohydride (NaBH4 ), methanol, and formate ions. This electrocatalyst synthesized by SFM was heat-treated (denoted as SFM TT), which improved its electrocatalytic activity during MOR. Later, Maiyalagan [165] reported that the addition of silicotungstic acid acted as a stabilizer for the PtRu particles on CNT support. The PtRu-supported CNT was prepared by microwave heating of an ethylene glycol (EG) solution of STA, H2 PtCl 6 .6H 2 O (as Pt precursor), and RuCl3 .xH2 O (as Ru precursor) with CNF suspended in the solution. The Pt and Ru precursors were loaded on CNF by conventional impregnation method. The results revealed that the PtRu nanoparticles are uniformly dispersed on carbon nanofiber support, with an average particle size of 3.9 nm enhanced the catalytic activity toward methanol electrooxidation. As a conclusion, the carbon nanotubes supporting material with high electronic conductivity and high surface area gives an advantage of better dispersion for the Pt or Pt alloys deposition. The higher the surface area of supporting material can reduce the agglomeration of metal particles on it, thus can produce better catalyst morphology for better fuel cell performance.
Mesoporous Carbon Support
Mesoporous carbon (MPC) support is another ideal candidate as an electrocatalyst support material in DMFC and fuel cell. Generally, mesoporous carbons are divided into two classes based on their structures which are ordered mesoporous carbons (OMCs), with highly ordered pore structure and uniform pore size, nonordered mesoporous carbons with irregular pores. Other than that, OPC can be produced by using high quality of SBA-15 silica and sucrose as carbon source template. To prepare the high quality of SBA-15 SBA-15 sample, triblock copolymer, EO20-PO70EO20 (Pluronic P123, BASF), as the surfactant and tetraethyl orthosilicate (TEOS, 98%, Acros) as the silica source are used, as reported by literature [166,167,168]. The synthesis of MPC starts from synthesis of SBA-15, followed by calcination process.
A well-dispersed and ultralow Pt catalyst (PtFe) supported on ordered mesoporous carbon (OMC) was prepared via a simple route and showed superior catalytic activity. The PtFe alloy with a size range of 3–5 nm was homogeneously dispersed on the CMS with a very high specific surface area of more than 1000 m 2 g -1 [169]. The incorporation of Fe was discussed in the previous section (“Performance of Various Types of Pt-Based Catalysts” section and “Performance of Pt-Based Alloys” section). The high specific surface area of mesoporous carbon support can be produced by carbonization process of a resorcinol-formaldehyde polymer with a cationic polyelectrolyte as a soft template [160]. The performance of Pt/MPC also related to the synthesis/preparation method as done by Kuppan and Selvam. Kuppan and Selvam [167] synthesized four type of Pt/mesoporous carbon by using different reducing agent which are NaBH4 , EG, hydrogen, and paraformaldehyde. From there, the Pt/mesoporous carbon synthesized using paraformaldehyde as reducing agent for showed highest current density. The highest in catalytic was attributed to the use of paraformaldehyde that gives the smallest Pt particle size (4.5 nm), and the highest ECSA (84 m 2 /g) belongs to Pt/mesoporous carbon.
王ら。 [161] synthesized a Pt@WC/OMC electrocatalyst composite, in which the composite was platinized using a pulsed microwave-assisted polyol technique. The OMC produced in this synthesis exhibited high surface area property. The Pt@WC/OMC electrocatalyst also showed high activity, desirable stability, and CO tolerance toward MOR. In another work done by Zhang et al. [170], the ordered CMS had a unique hierarchical nanostructure (with a 3-D structure) with ordered large mesopores and macropores that facilitated the dispersion of Pt nanoparticles and rapid mass transport during the reactions.
To maximize the use of Pt particles, the support materials should have uniform dispersion, high utilization efficiency, and desirable activity and stability. Moreover, the good supporting materials must be suitable for surface chemistry, high loading of Pt dispersion, and some functional roles. Additionally, based on the previous studies as discussed above, the ordered mesoporous carbons with large pore sizes are highly desirable for fast mass transfer and, thus, enhance the catalytic activity especially in the reaction involve large reactants molecules.
Carbon Black
Carbon black (CB) is one of the commercial carbon support that has been used till now. There are many types of CB such as Vulcan XC-72, Black Pearl 2000, Denka Black, Shawinigan Black, Ketjen EC-300J, etc. [171, 172]. CB is commonly used as a carbon support material for electrocatalysts because it possesses high porosity properties, which make it suitable as a potential support material for the catalyst layer in PEMFCs and DMFCs as reported in provided literatures [173,174,175,176,177,178,179,180]. The comparison of the several carbon black support was reported by Wang et al. [181] who investigated the effect on DMFC performance using several types of carbon black such as Vulcan XC-72R, Ketjen Black EC 300J, and Black Pearls 2000 carbon black as additives/support for the Pt cathode catalyst. From the experiments, the results showed that Ketjen Black EC 300J was the most useful carbon support for increasing the electrochemical surface area and DMFC performance of the cathode catalyst.
Nowadays, CB is commercial carbon support for many fuel cell systems. Generally, it is used for the comparison with new or modified catalyst [125]. The following Table 3 summarizes the commercial carbon black and its properties for fuel cell application. There are so many modifications among carbon support materials and development of new carbon support for enhance fuel cell performance; however, commercial carbon black still is used in many fuel cell applications especially for the comparison with new or modified catalyst.
<図> 図>Carbon Nanocoils
Celorrio et al. [182] proposed carbon nanocoils (CNCs) as a PtRu support in their experiment, indicating that the electrocatalyst performance was strongly dependent on the synthesis method. CNC-supported electrocatalysts showed better electrochemical behavior than E-TEK electrocatalysts, and better electrocatalytic behaviors toward CO and methanol oxidation were achieved using CNC as a support material [182]. Sevilla et al. obtained highly graphitic CNCs from the catalytic graphitization of carbon spherules via the hydrothermal treatment of different saccharides which are sucrose, glucose, and starch [183]. They demonstrated that the high electrocatalytic activity of the CNCs is due to the combination of good electrical conductivity of their graphitic structure and high porosity property, which allows much less diffusional resistance of reactants/products. Two years later, Sevilla et al. [184] reported highly dispersed Pt nanoparticles on graphitic CNCs with diameters in the range of 3.0–3.3 nm and a very fine particle size distribution. The electrocatalyst possessed large active Pt surface area (up to 85 m 2 g -1 Pt), high catalytic activity toward MOR (up to 201 A g −1 Pt), and high resistance against oxidation, which was noticeably greater than that of the Pt/Vulcan electrocatalyst. Celorrio et al. [185] obtained Pt/CNC electrocatalysts via the impregnation method, which showed that a combination of Pt and CNCs facilitated the CO oxidation process.
Conductive Polymer Supports
崔ほか[186] synthesized PtRu alloy nanoparticles with two types of conducting polymers, i.e., poly(N -vinyl carbazole) and poly(9-(4-vinyl-phenyl)carbazole), as the anode electrodes. Electrochemical and DMFC tests showed that these nanocomposite electrocatalysts were beneficial in a DMFC system, but their catalytic performance was still lower than that of a carbon supported electrode. Thus, they suggested that higher electrical conductivity of the polymer and lower catalyst loss are required in nanocomposite electrodes to achieve better performance in a DMFC.崔ほか[171] and Kim et al. [172] prepared polyaniline (PANi) as a support material for PtRu catalyst in a DMFC system. PANi is a group of conductive polymers with high electronic conductivity and a methanol oxidation current similar to that of carbon-supported PtRu catalyst. Then, Kim et al. [172] conducted catalytic tests to compare PtRu/PANi support with PtRu/carbon support, showing that the enhanced catalytic activity of PtRu/PANi was due to (i) the high electrical conductivity of the polyaniline support, (ii) the increase of electrochemical surface area of the prepared electrocatalyst, and (iii) the higher ion diffusion behavior. In another study, Amani et al. [74] synthesized PtSn supported by C-PANI as an electrocatalyst with different Pt:Sn atomic ratios using the impregnation method. The PtSn/C-PANI electrocatalyst with a ratio of 30:70 showed outstanding performance in the methanol electrooxidation, and the current density was approximately 40% higher than PtRu/C and 50% higher than Pt/C-PANi. The CO tolerance and stability were improved compared to that of PtRu/C, and the methanol crossover was reduced. Yaldagard et al. [173] studied the electrocatalytic performance of Pt/PANi/WC/C electrocatalyst for methanol electrooxidation (MOR) and oxygen electro-reduction (ORR), and it exhibited higher MOR activity, high CO resistance, and improved stability compared to Pt/C electrocatalyst in the presence of methanol.
ウーら[174] presented polypyrrole nanowire networks (PPNNs) as the anodic microporous layers (MPLs) of passive DMFC. In passive DMFC system, the novel MPL achieved a 28.3% increase in the power density from 33.9 to 43.5 mW cm −2 compared with the conventional layer with a similar PtRu (1:1). The high performance was due to the presence of PPNNs, which expressively improved the catalyst utilization and mass transfer of methanol on the anode. Besides, Selvaraj and Alagar [175] prepared Pt-Ru nanoparticle-decorated polypyrrole/multiwalled carbon nanotubes (Ppy/CNT) via the in situ polymerization of Ppy on CNTs containing ammonium peroxydisulphate (NH4 )S2 O 8 as an oxidizing agent at the temperature range of 0–5 °C, followed by deposition of Pt particles on PPy-CNT composite films via chemical reduction to produce Pt/PPy-CNT. It was found that the PtRu particles deposited on PPy–CNT composite films exhibited higher catalytic activity and stability toward MOR compared to Pt/PPy-CNT. So far, the investigation on polymer as supporting materials is not much as carbon support materials. From aspect as supporting materials, the performance of polymer support was not good/excellent as carbon support. Further studies are needed in the future for better electrocatalytic activity and DMFC performance.
Problems and Limitations of Using Pt for DMFC Systems
There are two major challenges in the development of new DMFC catalysts:(i) performance, including the catalytic activity, reliability, and durability; and (ii) catalyst cost reduction. Two major problems arise in DMFC when using pure Pt alone as the anode catalysts:(1) slower kinetics oxidation of methanol, even on some state-of-the-art anode catalysts, and methanol crossover through the membrane, which not only lowers cathode performance but also reduces fuel efficiency. To develop successful fuel cell technology, including DMFC technology, new catalysts must be investigated to improve the performance and reduce the cost. Reduction of the catalyst cost remains a major challenge. Currently, platinum is one of the most effective electrocatalysts for DMFC due to its high catalytic activity for methanol oxidation, but because it is a precious metal, platinum usage is challenging and limited [176, 177]. Therefore, many scientists have attempted to find materials that can behave like Pt catalysts. One problem with the MOR in DMFCs is that CO is produced as an intermediate reaction product when using Pt catalyst and has strong binding energy on platinum particles, poisoning the active sites of the platinum surface area [58]. Therefore, CO must be removed by oxidizing it from the Pt surface using another material with high resistance to CO poisoning. For example, Hwu et al. proposed Pt-modified WC catalyst that has remarkable resistance to CO poisoning [178]. On the other hand, they also suggested that CO tolerance originates from the lower CO desorption temperature on pure and Pt-modified WC compared to pure Pt.
There are many solutions that can be applied to reduce the cost of Pt, overcome or minimize the formation of CO species during methanol oxidation, and increase the kinetics of methanol oxidation, such as alloying with other metals or transition metals, the incorporation of metals, metal nitrides, and metal oxides and the use of carbon supports as discussed in this paper. However, to overcome this problem, we need to understand the formation of CO on Pt sites particle, and understanding of the mechanism of the anode reaction in DMFCs. Unfortunately, it has limited amount of mechanistic insight to be studied, because this reactions involve complex mechanism path with many possible intermediate molecules and also competing reaction pathways [179]. For Pt catalytic mechanism, it has been suggested by a direct reaction path. Unfortunately, the use of Pt on other metals has limited mechanistic information available. Figure 10 represents the reaction path for methanol electrooxidation and their possible intermediates molecules formed during the process. Black arrows show direct path, while green arrows show the indirect mechanism for CO2 formation as a final product. In the direct mechanism, the reaction path does not involve a CO intermediate, and CO2 is formed directly from methanol. In contrast, indirect mechanism forming a CO intermediate molecule and subsequently it is oxidized to CO2 製品。 Notably, CO is the most stable molecule of all the intermediates on Pt during MOR. The stability of CO causes it to be a main reason for the extensive CO poisoning problem that is often found on Pt catalyst.
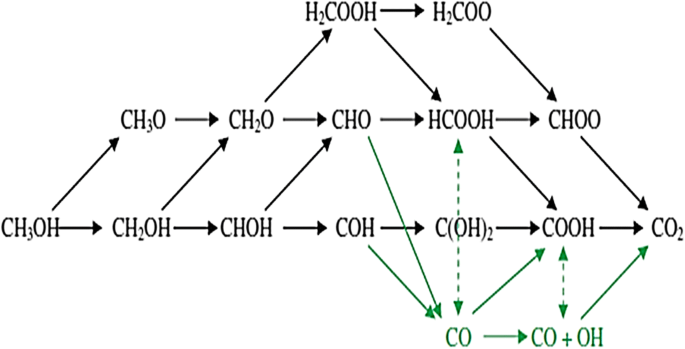
Schematic of the reaction paths and possible intermediates molecules considered in methanol electrooxidation [237]
First step in the mechanism of methanol decomposition reaction on Pt is the activation of methanol molecule. It can take place via hydrogen abstraction from either the carbon or the oxygen atoms. Further step, hydrogen abstraction creates formaldehyde (CH2 O) or hydroxymethylene (CHOH), followed by formyl (CHO) or COH. In the direct mechanism, instead of stripping off the final hydrogen from CHO or COH molecule to CO, a water molecule will release a proton/electron pair and resulting to OH species that can further bind with the carbonaceous species to form dihydroxycarbene (C(OH)2 ) or formic acid (HCOOH). This step is called hydroxyl addition process. The next step is followed by dehydrogenation to form either formate (HCOO) or carboxyl (COOH) molecule, with subsequent dehydrogenation to form CO2 as the final product of reaction. In addition, an alternative direct mechanism involve the stripping of a proton/electron pair from water and addition of the resulting hydroxyl to CH2 O, subsequently to H2 COOH, which then undergoes dehydrogenation to form HCOOH or dioxymethylene (Hs COO). The Hs COO molecule can then undergoes dehydrogenation to HCOO and finally to CO2 。 Besides, in the indirect mechanism, CHO or COH species are directly dehydrogenated to CO. Water is dissociated separately on the surface to form OH, and the two surface species react together to form CO2 gas in a way similar to the water-gas-shift reaction [187]. This indirect mechanism occurs because less energy is required to form CO than CO2 。 Strong adsorbed CO intermediate form on the Pt surface sites revealed a major problem at the anode site of DMFC. Formation of this intermediate species can cause deactivation Pt catalyst. Furthermore, the rate of kinetic methanol oxidation for DMFC is slower. Therefore, to increase the resistance of Pt catalyst to CO poisoning on the electrodes, Pt alloy or hybrids, such as PtRu, PtSn, PtMO, PtPb, PtFe, PtCo, PtNi, PtRuOs, PtRuMo, PtRuSn, PtRuNi, etc. (as mentioned and discussed in “Performance of various types of Pt-based catalysts” section), are usually employed as electrocatalyst materials on DMFC anodes. Addition/incorporation of these alloys to Pt can prevent the adoption of CO on Pt surface by decreasing the oxidation overpotential of the anode [84].
Conclusion and Prospects
Great progress has been made in recent years in the development and optimization of new catalysts using Pt-based catalysts and carbon and conductive polymer supports for DMFC anode catalyst. Some new carbon materials, such as nano- or mesostructured carbons, have been demonstrated as highly potential catalyst support materials, although their applications face challenges in terms of synthesis, metal loading, and electrode preparation. The combination of platinum as the best metal catalyst for DMFC and an excellent carbon support could produce breakthroughs in the investigation of a new DMFC anode catalyst in the future. Since platinum is an expensive metal, it is necessary to reduce the amount of Pt used in the electrocatalyst. Therefore, this paper presented more than 100 studies on the electrocatalytic activity and performance related to Pt-based electrocatalysts and various carbon and conductive polymer supports. The main problems related to the platinum electrocatalyst, such as carbon monoxide formation during the methanol oxidation reaction and the poor kinetics of methanol oxidation, could be overcome using additional materials and various supports, as reported in the research presented in this paper.
Many studies conducted in the recent years to reduce the loading amount of Pt catalyst and to increase the percentage utilization efficiency, and hence, enhance the electrocatalytic activity of Pt toward the oxygen reduction reaction (ORR) and methanol electrooxidation reaction (MOR), were discussed in this paper. Pt has been alloyed with many transition metals such as Fe, Co, Ni, Ir, Ru, Rh, and Pd, resulting in higher catalytic activity for the DMFC system. The incorporation of these materials also resulted in good dispersion on the carbon and polymer supports, which showed higher performance in the DMFC test compared to the use of Pt metal alone. Various carbon support sources, namely activated carbon (AC), carbon black (CB), multiwall carbon nanotubes (MWCNTs), carbon nanofibers (CNFs), carbon nanotubes (CNTs), graphene, and conductive polymer supports, have been used with Pt-based catalysts to improve their catalytic performance. Additionally, Pt-based alloy catalysts have been designed as hollow mesoporous PtNi, nanowire PtRu, and nanodendritic PtRh, which showed improved electrocatalytic activity and superior electrocatalytic performance. Meanwhile, 3-D Pt/C/graphene aerogel demonstrated enhanced stability toward methanol electrooxidation. The work performed by researchers showed that the electrocatalytic activities of nanoparticles Pt alloy catalysts depend on several factors such as the synthesis method, condition of experiments (such as temperature and pH), alloy composition/ratio, precursors, and thermal treatment. For the future study, it should be extended to the optimization of the geometry and structure of previous studies that revealed active Pt alloys can increase their electrocatalytic activity and stability and the application of support materials for fuel cell applications. For example, current research that have been done by Liu et al. 2017 [188] shows the excellent performance of platinum. From theoretical calculations, it revealed that the main effective sites on platinum single atom electrocatalysts are single-pyridinic-nitrogen-atom-anchored single-platinum-atom centers, which ascribed to the tolerant CO in MOR. They also suggested that carbon black supported used together with Pt single atom is effective in cost, efficient, and durable electrocatalyst for fuel cell application. According to the above study, herein, we can conclude that the modification on structure and morphology of precious metal such as platinum could also remarkably increase the performance of electrocatalyst, but in the same time can reduce the overall cost of fuel cell for commercialization.
To improve the morphologies of Pt and Pt alloys, carbon support material also need further study. Nanoporous metals become an interesting part of catalyst to be studied for fuel cell application. It is determined very suitable for fuel cell catalysts because they possess high surface area, three-dimensional (3D) network structures with adjustable ligament/pore sizes suitable for mass transport, and electron conduction. Around 2017, Li et al. successfully carried out modification on Pt-Pd-Au trimetallic surface as cathode for oxygen reduction reaction [189]. The surface evolution of 3-D Pt-Pd-Au trimetallic greatly enhanced the ORR activity and highly stable as ORR catalyst. The modification of PtNi alloy also done by Li et al. 2016 [190] showed ultrafine jagged platinum nanowire with highly large ECSA that exhibits enhanced mass activity of ~ 50 times higher than state-of-the-art commercial Pt/C catalyst, while Bu et al. 2016 [191] reported highly uniform PtPb/Pt core/shell nanoplate with biaxially strain extremely active, stable for anodic oxidation reactions, and great performance compared to commercial Pt/C in both methanol oxidation reaction (MOR) and ethanol oxidation reaction (EOR). Since the nanostructured platinum becomes an efficient catalyst for fuel cells as well as various industrial chemical reactions. Thus, these modifications on surface of Pt particles electrocatalysts could also to be applied in MOR for future DMFC.
On the other hand, to reduce the consumption of the Pt catalysts, the modification of the carbon support is also another useful way. This not only improves the transport capacity of protons but also reduces the usage of Nafion, which can cut the cost of the fuel cell. Moreover, with regards to the carbon support for the ORR catalysis, the hydrophobic carbon support material is required to allow water (product) to be quickly removed from the catalyst surface sites, and oxygen (reactant) to access the active sites. In contrast, the MOR catalysis requires a certain degree of hydrophilic carbon support. It can be achieved by the modification of the carbon support materials. By combination of modified carbon support materials and development of new carbon support with Pt metal catalyst, it is possible to get an ideal electrocatalysts for direct methanol fuel cell technology. Combination of Pt metal with varied carbon supports with different specific surface areas, structures, pore sizes, electronic properties, and morphologies could be great catalyst to be studied for future DMFC.
Carbon support also influence the overall performance for DMFC. Vulcan XC-72R, which is a commercial carbon support, has a large surface area, appropriate particle size, and good electrical conductivity for good support. However, in the process of depositing metal particle on these support with loading of 40% or more, the particle size of metal increased quickly, which is a disadvantage for DMFC, because a higher metal loading is used to give a better performance. In addition, multiwalled carbon nanotubes (MWCNTs) and carbon nanofibers (CNFs) with relatively smaller surface area, large diameter, and high aspect ratio could be very difficult to deposit a catalyst with high loading metal (40% and more). Therefore, modification of MWCNTs and CNFs support must be done to improve its surface area, surface functional groups, and reduce the wall thickness to achieve outstanding performance for direct methanol fuel cell even though high loading metal catalyst is consumed. As well, a great and important part to be further studied in DMFC system is about the anode and cathode catalyst preparation approaches.
略語
- CB:
-
Carbon black
- CH3 O:
-
methoxy group
- CNC:
-
Carbon nano cage
- CNF:
-
Carbon nano fiber
- CNT:
-
Carbon nano tube
- Co:
-
Cobalt
- Co:
-
Cobalt
- CO:
-
Monoxide molecules
- CO2 :
-
Carbon dioxide
- DMFC:
-
Direct methanol fuel cell
- FC:
-
Fuel cell
- Fe:
-
鉄
- MOR:
-
Methanol oxidation reaction
- MPC:
-
Mesoporous carbon
- MWCNT:
-
Multi wall carbon nanotube
- Ni:
-
ニッケル
- OMC:
-
Ordered mesoporous carbon
- ORR:
-
酸素還元反応
- PANi:
-
Polyaniline
- PEMFC:
-
Proton exchange membrane fuel cell
- Ppy:
-
Polypyrrole
- Pt:
-
Platinum
- Pt/MWCNT:
-
Platinum-supported MWCNT
- Pt-Ru/MWCNT:
-
Platinum-ruthenium-supported MWCNT
- Rh:
-
Rhodium
- Ru:
-
Ruthenium
- Sn:
-
Sternum
- SOFC:
-
Solid oxide fuel cell
- SWCNT:
-
Single-wall carbon nanotube
- TMN:
-
Transition metal nitride
ナノマテリアル
- 改善された診断および治療用途のための多機能金ナノ粒子:レビュー
- 合成および生物医学的応用のための蛍光ナノ材料の進歩と挑戦
- スーパーキャパシター用途向けのグラフェンおよびポリマー複合材料:レビュー
- エレクトロスピニング法による直接メタノール燃料電池用の新しい複合Tio2カーボンナノファイバー陽極触媒担体の製造と特性化
- グリセロール燃料電池の電気酸化用の新しい陽極PdAu / VGCNF触媒の性能の向上
- スーパーキャパシター用途の電極としてのグラフェン/ WO3およびグラフェン/ CeOx構造の評価
- 直接メタノール燃料電池用の新しい陽極触媒のサポート:特性評価と単一セル性能
- 金ナノクラスターの生物医学的応用:最近の発展と将来の展望
- レビュー:油水分離用の多孔質金属フィルターと膜
- 蛍光ネオ糖タンパク質金ナノクラスター:合成と植物レクチンセンシングおよび細胞イメージングへの応用
- ソルベイは、海洋石油およびガス用途向けの高性能カーボンファイバーテープを発売