Ru / SnxTi1-xO2ディーゼル酸化触媒の新しいワンステップ水熱調製とその低温性能
要約
ルチルSn x Ti 1- x O 2 ( x =0、0.33、0.5、0.67、1)固溶体は、チタン酸テトラブチルと塩化スズ(IV)五水和物を原料としたワンステップ水熱法で合成しました。一連のRu / Sn x Ti 1- x O 2 次に、RuCl 3 での含浸プロセスによって調製されました。 COとC 3 のパフォーマンスと安定性を調査する H 8 酸化。これらの触媒は、XRD、N 2 によって特徴づけられました。 吸着-脱着、FT-IR、TEM、XPS、H 2 -TPR、およびO 2 -TPDテクニック。 Ru / Sn x の低温接触酸化性能と安定性に及ぼすSn / Tiモル比と熱水条件の影響 Ti 1- x O 2 調査されました。結果は、Ru / Sn 0.67 Ti 0.33 O 2 触媒は低温で優れた活性と安定性を示した。 CO変換は180°Cで50%、240°Cで90%に達しました。その上、C 3 H 8 変換は320°Cで50%に達し、C 3 の完全な変換です。 H 8 500°Cで実現され、12時間の触媒反応後に失活は発生しません。 Ru / Sn 0.67 の優れた低温活性と安定性 Ti 0.33 O 2 以下の要因によるものです。まず、XRDの結果は、Sn 4+ TiO 2 の格子にうまく導入されました Ti 4+ を置き換える 均質な固溶体を形成する(–Sn 4+ を含む –o–Ti 4+ –種)、これはTEMおよびN 2 と一致していました 吸着-脱着の結果。 Snの導入はアナターゼ結晶の成長を抑制し、ルチル相の形成を促進する可能性があり、この相転移は触媒の低温活性を改善するのに役立ちました。第二に、TEM画像は超微細Ruナノ粒子(〜5 nm)がSn 0.67 に分散していることを示しました Ti 0.33 O 2 サポート、Sn x の形成を示唆 Ti 1- x O 2 固溶体はRu粒子の分散に有益でした。
背景
ディーゼルエンジンは、燃料消費量が少なく、熱効率が高く、安定性が高いという利点があるため、輸送、鉱業、エンジニアリング機械の分野で広く使用されています[1]。ただし、一酸化炭素(CO)、未燃炭化水素(HC)、さまざまな窒素酸化物(NO x )、およびディーゼル車の排気ガス中の粒子状物質(PM)は、生態系環境と人間の健康に深刻な脅威をもたらしています[2、3]。さらに、厳しい環境法および規制は、ディーゼル排出制御技術の最近の進歩を推進しています。ディーゼル酸化触媒(DOC)、選択的触媒還元(SCR)、および触媒ディーゼルパティキュレートフィルター(DPF)で構成される統合排気後処理システムは、ディーゼル排気を浄化するために広く使用されています。後処理システムにおけるDOCの機能は、CO、HC、およびNOをCO 2 に変換することです。 、H 2 O、およびNO 2 、NO 2 その後のde-NO x の原料として使用されます SCR反応を促進する反応。さらに、可溶性有機画分(SOF)を酸化して、PM排出量を減らすこともできます。ディーゼル車のコールドスタート時のHCの不完全燃焼により、HCの過剰排出が発生します。したがって、触媒は低温で急速に発火する必要があります[4]。現在、炭素材料または酸化物(TiO 2 など)に担持された貴金属触媒(Pt、Pd、Rhなど) 、Al 2 O 3 、CeO 2 、およびZrO 2 )は、CO、NO、およびHCの触媒酸化に優れた性能を備えた市販のディーゼル酸化触媒です。ただし、市販の触媒には、熱安定性が低い、COによる自己抑制が強い、コストが高いなどの欠点があります[5]。
RuとRuO x 触媒は、CO [6]、メタン[7]、およびクロロベンゼン[8]の酸化に広く適用されています。重要なことに、Ru触媒は優れた低温活性と耐毒性を備えています[8、9、10、11]。しかし、RuとRuO x 焼結が容易であるため、活性部位の露出が減少します[12]。したがって、Ru触媒は、焼結を防ぎ、触媒活性を向上させるために、担体に担持する必要があります。
TiO 2 ディーゼル排気ガスの浄化に広く使用されています。 RuO x およびルチル相TiO 2 同様の格子定数を持ち、ルチル型TiO 2 Ru / TiO 2 で 触媒はRuO x の安定化に重要な役割を果たします アナターゼでサポートされたRuO x と比較した焼成プロセス中の粒子 触媒。したがって、RuO x TiO 2 の表面に高度に分散させることができます 。さらに、RuO x の間には相乗効果があります およびTiO 2 、Ru / TiO 2 の酸化還元能力を向上させるのに有益です [13、14、15、16、17、18]。熱安定性、活性成分の分散、アナターゼのルチル相への変換をさらに改善するために、多くの研究でSn 4+ が導入されています。 TiO 2 に Sn x を形成する Ti 1- x O 2 固溶体。 Huang etal。 [16]は、Sn 4+ の導入が TiO 2 に 格子はCuO / Ti x の安定性を改善する可能性があります Sn 1- x O 2 触媒とCuOの分散。 Bai et al。[17]は、Sn 4+ を示しました TiO 2 の熱安定性を大幅に改善 。 Mehraz etal。 [18]ドーピングSn 4+ が見つかりました TiO 2 の相転移を促進 アナターゼからルチルまで。
これまでの研究では、共沈法、ゾルゲル法、固相反応によるディーゼル酸化触媒の調製に焦点が当てられてきました[5、6、15、19、20]。ヤンら。 [19] Pt / TiO 2 を準備しました 共沈法による触媒作用により、COとC 3 H 6 232°Cでのみ50%に達します。 Li etal。 [15]合成されたTiO 2 –SnO 2 ゾルゲル法によるナノコンポジットであり、TiO 2 の変換が示唆された –SnO 2 COへの変換は260°Cで90%でした。シャリフら。 [6]準備されたRu / [Ca 24 Al 28 O 64 ] 4+ (O 2- ) 2 固相反応により、Ru / [Ca 24 Al 28 O 64 ] 4+ (O 2- ) 2 Ruの分散が低いため、COへの変換は240°Cでわずか82%でした。したがって、ディーゼル酸化触媒の低温活性に残る重大な課題があり、ディーゼルコールドスタートで発生するCOおよびHCを除去するために多くの努力が依然として必要です。さらに、現在の研究[8、16、19、21、22]は、主に共沈法とゾルゲル法によるDOC触媒の調製に焦点を当てています。これは、粒子サイズが小さいですが、サンプルの結晶化度が低く、複数結晶相;さらに、その後の共沈法による混合物の熱処理プロセスが必要である。従来の煆焼プロセスや、低温触媒活性を向上させる可能性のある触媒の固い凝集の形成を回避するために、準備プロセスで水熱処理が採用されています[23]。ただし、ワンステップ水熱法に関する体系的かつ包括的な研究は不足しています[24、25]。
したがって、RuO x Sn 4+ でサポートされているパーティクル -変更されたTiO 2 ワンステップ水熱法により、低温活性と安定性が期待できる優れたCOおよびHC酸化触媒でした。一連のSn x Ti 1- x O 2 ( x =0、0.33、0.5、0.67、1)固溶体はワンステップ水熱法で調製しました。 Ru / Sn x Ti 1- x O 2 次に、Sn x を含浸させて調製しました。 Ti 1- x O 2 RuCl 3 を使用 COとC 3 を酸化する H 8 。熱水温度、熱水時間、煆焼温度、およびRu / Sn x のSn / Tiのモル比の影響 Ti 1- x O 2 低温活性と安定性を改善するために触媒が調査されました。
メソッド
資料
塩化スズ(IV)五水和物(SnCl 4 ・5H 2 O)はGuangdong Kehua Stock Corporationから購入した、チタン酸テトラブチル(C 16 H 36 O 4 Ti)はTianjin Kemiou Chemical Reagent Factoryから購入し、塩化ルテニウム(III)、無水RuCl 3 、(37%Ru w / w)はアラジンから購入しました。
触媒の準備
Sn x Ti 1- x O 2 固溶体はワンステップ水熱法で調製した。一定量のSnCl 4 ・5H 2 OおよびC 16 H 36 O 4 Tiをそれぞれ200mLの脱イオン水と10mLの無水エタノールに溶解しました。次に、C 16 H 36 O 4 Tiエタノール溶液とSnCl 4 ・5H 2 O水溶液を室温で0.5時間撹拌しながら混合した。均一な混合物を、180°Cで24時間250mLオートクレーブに入れました。その後、混合溶液を遠心分離し、Cl - の残留物がなくなるまで、脱イオン水とエタノールで数回洗浄しました。 、次にオーブンで80℃で一晩乾燥させた。続いて、Sn x という名前の淡黄色の固体生成物が得られた。 Ti 1- x O 2 。 SnO 2 およびTiO 2 それぞれ同様の準備方法で得られました。
Ru / Sn x Ti 1- x O 2 触媒はSn x の含浸によって調製されました Ti 1- x O 2 1.0 wt。%のRuCl 3 を含む水溶液で 。これらのサンプルを2時間超音波攪拌し、80°Cで12時間乾燥させた後、400°Cで3時間焼成しました(加熱速度は3°C /分)。得られた粉末をRu / Sn x と名付けた。 Ti 1- x O 2 。
触媒パフォーマンス
触媒の活性は、電気ヒーターを備えた固定床石英反応器で評価された。模擬反応ガスには、3000 ppmのCO、600ppmのC 3 の混合物が含まれていました。 H 8 、600 ppm NO、50 ppm SO 2 、7%O 2 、およびN 2 60,000 mLg -1 のガス空間速度でバランスをとる h -1 。ガス流量はマスフローコントローラーによって調整されました。固定床の温度は、中央のチャネルの中央に配置された0.5 mmKの熱電対によってテストされました。アウトレットCOおよびC 3 H 8 KM9106煙道ガス分析装置(Kane International Limited、英国)によって測定されました。変換( X )COおよびC 3 H 8 次の式を使用して計算されました:
$$ X =\ frac {c _ {\ mathrm {in}}-{c} _ {\ mathrm {out}}} {c _ {\ mathrm {in}}} \ times 100 \%$$ここで c in COまたはC 3 の初期濃度です。 H 8 および c out COまたはC 3 の瞬間です H 8 反応温度で; T 50 低温触媒活性指数として表されます。
触媒の特性評価
サンプルのX線回折(XRD)パターンは、CuKα線(0.15418 nm)を使用した高温チャンバーを備えたBRUKER D8ADVANCE回折計でのパワーX線回折によって実行されました。 X線管は40kV×40mAの線源電力で操作されました。
Brunauer-Emmett-Teller(BET)の表面積は、MicromeriticsASAP2020吸着装置で77Kでの窒素吸着によってテストされました。比表面積と細孔分布は、それぞれBET法とBJH法によって計算されました。これらのサンプルは、各分析の前に、真空下で300°Cで4時間脱気しました。
フーリエ変換赤外(FT-IR)分光法は、Nicoletis5分光計を使用して4.0cm -1 のスペクトル分解能で調べました。 。粉末を自立型ウェーハ(約15 mg、直径12 mm)にプレスしました。ウェーハはN 2 で前処理されました 300°Cで1時間。周囲温度に冷却した後、サンプルのスペクトルを記録しました。
これらのサンプルの透過型電子顕微鏡(TEM)画像は、Tecnai G2F20装置によって加速電圧200kVで取得されました。サンプルは粉砕され、エタノールに分散され、観察前にカーボンコーティングされた銅グリッド上に堆積されました。
X線光電子分光法(XPS)分析は、ESCALAB250Xi分光計で、15 kWの加速電力で単色AlKα放射線(1486.6 eV)を使用して実行されました。得られたサンプルスペクトルは、内部参照標準としてC1s(284.6 eV)を使用して補正されました。
H 2 -温度プログラムによる還元(H 2 -TPR)実験は、H 2 を備えた熱伝導度検出器(TCD)に接続された石英反応器で実行されました。 (6.9%vol。%)-還元剤としてのAr混合物(30 mL / min)。反応の前に、サンプル(50 mg)をN 2 で前処理しました。 300°Cで1時間、その後室温まで冷却します。 TPRは、室温から目標温度まで10°C /分の速度で開始しました。
温度プログラムされた酸素脱着(O 2 -TPD)実験は、H 2 と同じデバイスを使用して実行されました。 -TPR。使用済み触媒(50 mg)は、Arを30 mL / minで流しながら、300°Cで1時間前処理しました。次に、O 2 の下で酸素吸着を行った。 –Ar混合物(20%O 2 巻%)500°Cで0.5時間。室温まで冷却した後、システムをAr(30 mL / min)で1時間パージしました。処理後、目標温度(10℃/分)まで上昇させました。
CO吸着のinsitu赤外分光法(IR)は、Nicolet 5700FT-IR分光計で4.0cm -1 のスペクトル分解能で収集されました。 。 CO吸着は、触媒の自立型ウェーハ(約15 mg)を露光し、商用制御環境チャンバー(HTC-3)にマウントすることによって実行されました。サンプルは、CO–Arの制御されたストリーム(COの10体積%)に5.0 mL / minの速度で40分間さらされました。スペクトルは、室温から300°Cまで10°C /分の速度でさまざまな目標温度で記録されました。
結果と考察
触媒活性と安定性
図1は、COとC 3 の触媒活性を示しています。 H 8 Ru / Sn x での酸化 Ti 1- x O 2 180°Cの熱水温度、24時間の熱水時間、および400°Cの煆焼温度の最適な調製条件(図S1、S2、およびS3)での触媒。 Ru / Sn x の触媒性能がわかる Ti 1- x O 2 触媒は最初に増加し、次に反応温度の上昇とともに安定化する傾向がありました。 Sn / Tiのモル比が2/1の場合、 T 50 Ru / Sn 0.67 の Ti 0.33 O 2 COとC 3 を酸化する H 8 はそれぞれ180°Cと320°Cであり、他のSn / Tiモル比よりも低い反応温度です。 COの変換は240°Cで90%に達し、C 3 の完全な変換 H 8 Ru / Sn 0.67 で500°Cで達成できます Ti 0.33 O 2 触媒。各サンプルの触媒性能は、図2に示すように、表面のRu原子に関して正規化され、ターンオーバー頻度(TOF)で表されます。Ru/ Sn 0.67 のTOF値 Ti 0.33 O 2 どの反応温度でも、すべてのサンプルの中で最高でした。これは、Sn 0.67 の表面に高度に分散したRuに起因します。 Ti 0.33 O 2 、および有効成分RuはキャリアSn 0.67 と強い相互作用を持っています Ti 0.33 O 2 [22、26]。シャリフら。 [6]は、Ru / [Ca 24 Al 28 O 64 ] 4+ (O 2- ) 2 COへの変換は240°Cでわずか82%でした。村山ほか[27]は、Au / Nb 2 の変換を報告しました O 5 およびAu / SiO 2 COに対する反応は、250°Cでそれぞれ55%と38%でした。他の文献[27、28]と比較して、Sn / Tiのモル比が2/1の場合、この研究では低温でより高いCO変換を達成できます。 Okal etal。 [29]は、 T 50 CH 4 の Ru / ZnAl 2 によって酸化 O 4 Ruの負荷がそれぞれ0.5wt。%、1.0 wt。%、4.5 wt。%の場合、触媒は480、500、540°Cでした。 Wilburn etal。 [30]は、 T 50 CH 4 の 0.3Pd–0.7Pt / γ–Al 2 での酸化 O 3 触媒は360°Cでした。 COおよびC 3 に対するさまざまな触媒の触媒活性 H 8 酸化を表S1と表S2に示します。 C 3 の完全な変換 H 8 この作業では、より低い温度で達成することができます。 Sn / Tiの最適なモル比は2/1であり、これはCOの活性と一致しています。上記の分析から、COとC 3 の変換は結論付けることができます。 H 8 Sn / Tiのモル比に大きく影響されます。 Sn / Tiのモル比が2/1の場合、 T 50 Ru / Sn 0.67 の Ti 0.33 O 2 COとC 3 H 8 それぞれ180°Cと320°Cです。反応温度が240°Cの場合、COの変換率は90%に達し、C 3 の完全な変換率に達する可能性があります。 H 8 反応温度が500°Cのときに達成できます。
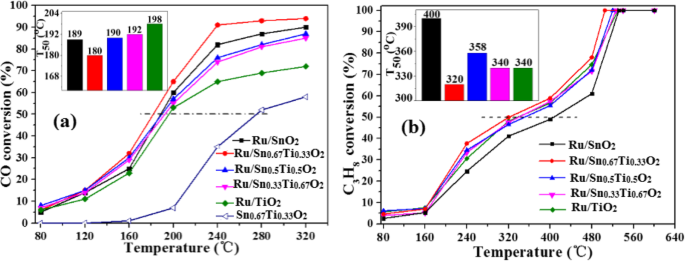
Ru / Sn x に対するさまざまなSn / Tiモル比の影響 Ti 1- x O 2 COの接触酸化( a )およびC 3 H 8 ( b )
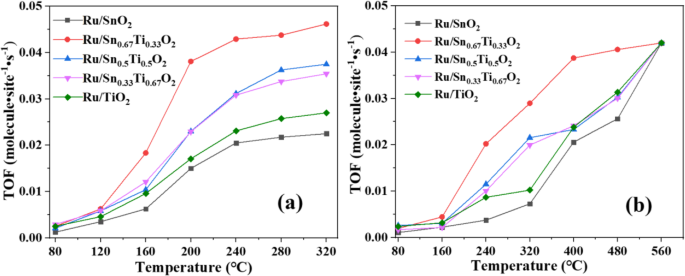
COの反応温度のTOF( a )およびC 3 H 8 ( b )さまざまな触媒による酸化
COとC 3 の安定性 H 8 図3で、180°Cの熱水温度、24時間の熱水時間、および400°Cの煆焼温度で調査しました(図S1、S2、およびS3)。 COの変換は240°Cで90%に達し、C 3 の完全な変換 H 8 500°Cで達成できます。興味深いことに、Ru / Sn 0.67 Ti 0.33 O 2 触媒は基本的に12時間の触媒反応後に不活性化されます。ただし、Ru / TiO 2 のアクティビティ およびRu / SnO 2 触媒は、COを酸化する時間の増加とともにわずかに減少しました。この現象は、Sn x の形成を示しています。 Ti 1- x O 2 固溶体は、触媒の活性を向上させるだけでなく、安定性を高めることもできます。 RuはSn 0.67 の表面に高度に分散していると考えられます。 Ti 0.33 O 2 ;有効成分Ruと担体Sn 0.67 の間には強い相互作用があります Ti 0.33 O 2 [26]。
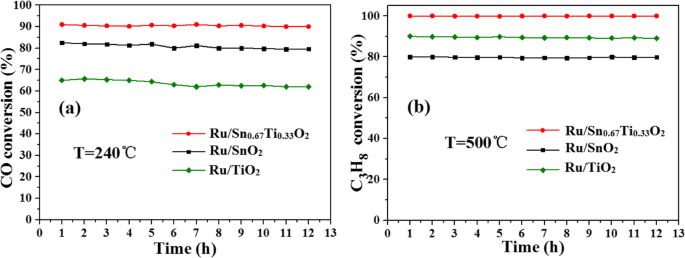
Ru / Sn x の安定性 Ti 1- x O 2 触媒CO( a )およびC 3 H 8 ( b )
触媒の特性評価
Sn x のテクスチャプロパティ Ti 1- x O 2 サポートとRu / Sn x Ti 1- x O 2 触媒
図4は、両方のSn x のXRDパターンを示しています。 Ti 1- x O 2 固溶体とRu / Sn x Ti 1- x O 2 触媒。アナターゼ構造の典型的なピークは、TiO 2 で観察されます。 (25.78°)およびRu / TiO 2 (25.67°)粒子サイズがそれぞれ約4nmと5.5nmのサンプル(表1)。アナターゼからルチルへの相転移は、Snの導入とともに現れました。 Ruの回折ピークは観察されません。これは、RuがSn x に高度に分散していることを示しています。 Ti 1- x O 2 表面またはXRD検出限界を超えています[31]。さらに、Sn x の回折ピーク Ti 1- x O 2 およびRu / Sn x Ti 1- x O 2 Sn含有量の増加に伴い、徐々に低い角度に移動します。これは、面間の間隔が d であることを示しています。 ブラッグの法則に従って増加します、2 d sin θ =nλ 。これは、正方晶の格子定数( a )の増加と一致しています。 および c )表1で、これはより大きなイオン半径Sn 4+ の置換に起因します。 (0.071 nm)Ti 4+ の場合 (0.068 nm)。結果は、Sn 4+ を示唆しています。 TiO 2 へのドーピングに成功しました 均一な格子を形成する(–Sn 4+ –o–Ti 4+ –)ルチル相構造を維持しながら固溶体を形成します。これは以前のいくつかの研究[5、18]と一致しています。
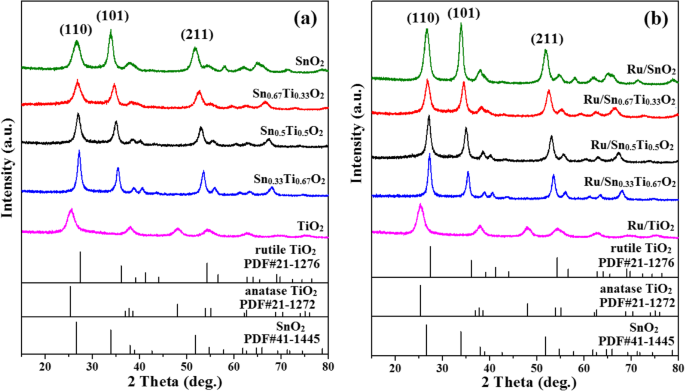
Sn x のXRDパターン Ti 1- x O 2 サポート( a )およびRu / Sn x Ti 1- x O 2 触媒( b )
サンプルのテクスチャプロパティを決定するには、N 2 吸着-脱着技術が使用されました。 N 2 これらのサンプルの吸脱着等温線と対応する細孔径分布曲線を図5に示します。N 2 SnO 2 の吸脱着等温線 明らかにタイプIIに属します。その他は、IUPAC分類による古典的なタイプIVであり、 p / p にH2複素ヒステリシスループを示します。 0 メソポーラス材料の一般的な特徴である0.4〜0.95の範囲(図5a、c)[17、32]。これらのメソ細孔の存在は、触媒の比表面積が大きい重要な理由です[33]。すべてのSn x Ti 1- x O 2 サポートとRu / Sn x Ti 1- x O 2 触媒は、小さなサイズの細孔(3〜8 nm)、特にSn 0.67 の狭い分布を示しました。 Ti 0.33 O 2 サポートとRu / Sn 0.67 Ti 0.33 O 2 細孔径が主に5nm付近に均一に分布している触媒(図5b、d)。この現象は、適切な量のSnが触媒表面の拡散係数を弱め、結晶子の凝集を間接的に妨げる可能性があることを示唆しています[17]。
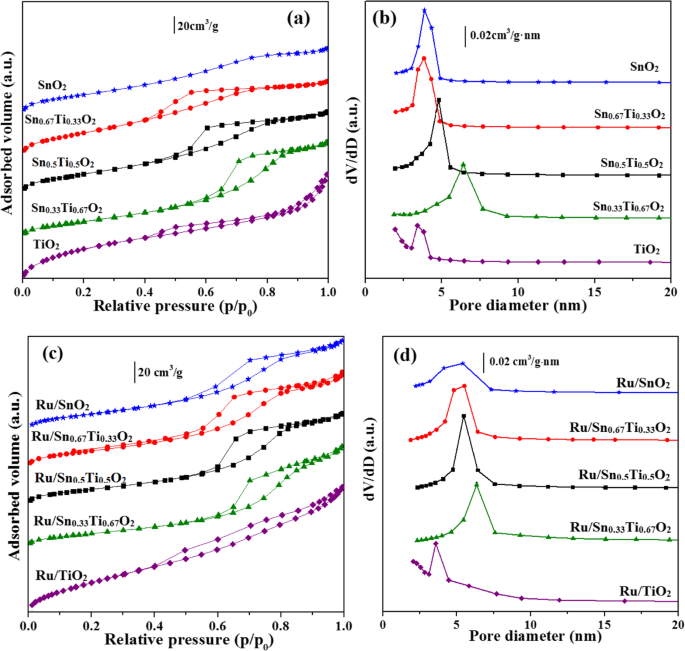
N 2 Sn x の吸脱着等温線 Ti 1- x O 2 ( a )およびRu / Sn x Ti 1- x O 2 ( c )Sn x の細孔径分布 Ti 1- x O 2 ( b )およびRu / Sn x Ti 1- x O 2 ( d )
Sn x のテクスチャプロパティ Ti 1- x O 2 サポートとRu / Sn x Ti 1- x O 2 触媒を表1に示します。比表面積と細孔分布は、BETおよびBJH法によって計算されました。 Sn 0.67 の比表面積と細孔容積の両方 Ti 0.33 O 2 156.5 m 2 g -1 および0.17cm 3 g -1 、 それぞれ。しかし、Ru / Sn 0.67 の比表面積と細孔容積の両方 Ti 0.33 O 2 触媒はSn 0.67 と比較して減少しています Ti 0.33 O 2 サポート。これは、RuがSn 0.67 にロードされたことを示します。 Ti 0.33 O 2 水面。さらに、Ru / Sn 0.67 Ti 0.33 O 2 高温焼成プロセス中に、触媒が焼結され、開いた細孔構造が崩壊して、詰まった細孔を形成します[31]。それにもかかわらず、Ru / Sn 0.67 Ti 0.33 O 2 それでもより大きな比表面積を維持します(83.3 m 2 g -1 )およびRu / Sn 0.33 などの他のルチルサンプルと比較して小さい細孔径(5.3 nm) Ti 0.67 O 2 、Ru / Sn 0.5 Ti 0.5 O 2 、およびRu / SnO 2 。
図6は、Sn x のFT-IRスペクトルを示しています。 Ti 1- x O 2 サポートとRu / Sn x Ti 1- x O 2 触媒。すべてのサンプルは、類似の波数位置で類似の振動ピークを示します。約3223.68cm -1 での吸着 これは、酸素空孔サイトに隣接する表面ヒドロキシル基によるものです[34、35]。 1501.86〜1618.18 cm -1 のバンド 水の角振動ピークに属します。格子酸素の対称伸縮振動ピークは1028.17cm -1 に現れます。 。 527.27–681.2 cm -1 のバンド TiO 2 の伸縮振動ピークに起因する可能性があります またはSnO 2 [34]。 Sn x との比較 Ti 1- x O 2 サポート、Ru / Sn x Ti 1- x O 2 スペクトルが広がり、有効成分RuがSn x をサポートしていることを示しています Ti 1- x O 2 相互作用があり、触媒の表面欠陥が発生します[36、37]。
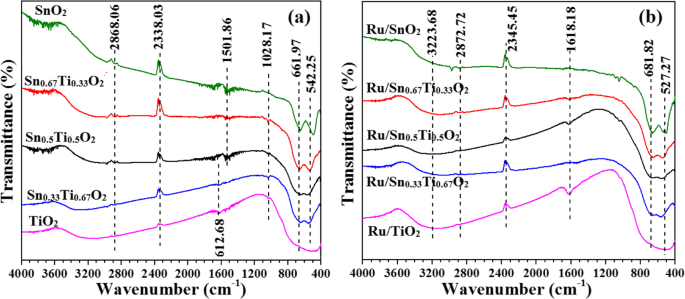
Sn x のFT-IRスペクトル Ti 1- x O 2 サポート( a )およびRu / Sn x Ti 1- x O 2 触媒( b )
触媒の形態
低解像度および高解像度のTEM、HRTEM画像、およびRu / Sn x の粒子サイズ分布 Ti 1- x O 2 図7a、d、g、j、およびmに示されているTEM画像の観察に基づいて、すべてのサンプルが不規則な形状と無秩序なメソポーラス構造を持つ明確な粒子で構成されていることがわかります。これは、ナノ粒子の凝集によって形成されます[38]。さらに、Ru / Sn 0.67 Ti 0.33 O 2 これらのサンプルの中で最小の粒子サイズのため、サンプルは最高の凝集度を持っています。 HRTEM画像(図7b、e、h、k、n)から、これらのサンプルの(110)面と互換性のある0.327nmの格子縞は1種類だけです。さらに、TiO 2 の格子縞が見つかります。 およびSnO 2 Sn 4+ に起因する観察されない TiO 2 の格子にうまくドープされた 均質なSn x を形成する Ti 1- x O 2 固溶体[39]。結果はXRDと一致しています。 Ru粒子サイズ分布(図7c、f、i、l、o)は、Ru粒子のおおよそのサイズが3〜20nmの範囲であることを示しています。 Sn 4+ の紹介 Ru粒子のサイズを効果的に縮小し、Sn x でより高い分散を実現できます。 Ti 1- x O 2 水面。他のサンプルと比較すると、Ru / Sn 0.5 のRu粒度分布 Ti 0.5 O 2 サンプルの幅が広く(<13 nm)、これは(–Sn 4+ 間の相互作用が原因である可能性があります –o–Ti 4+ –)種とRu [26]。 Ru / Sn 0.67 Ti 0.33 O 2 触媒は、すべてのサンプルの中で、Ruの分散が良く、粒子サイズが小さい(5.49 nm)。
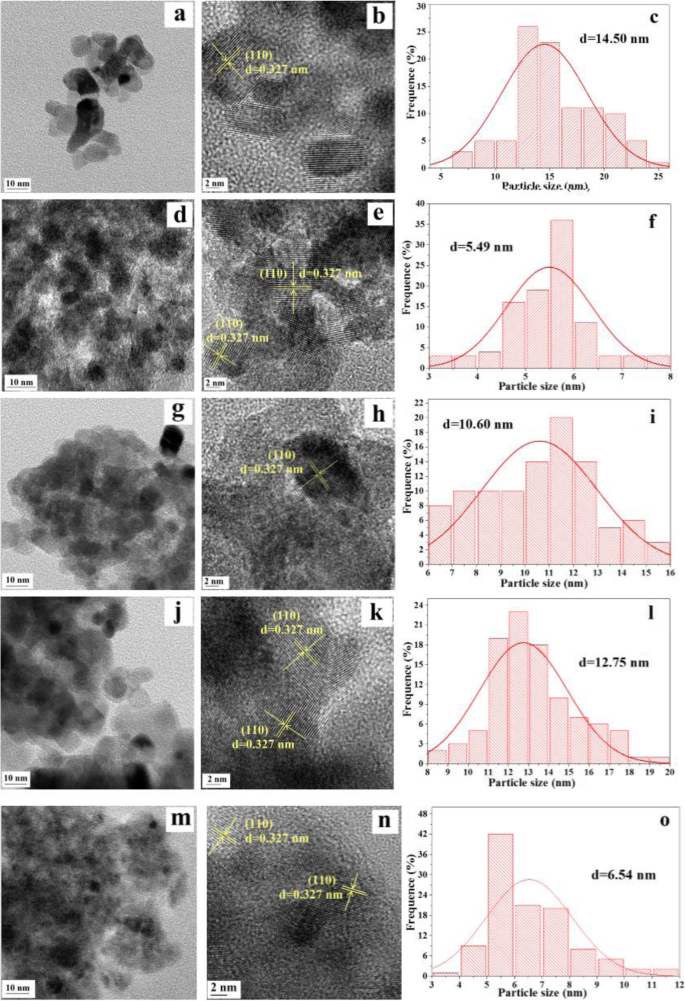
TEM、HRTEM画像、および a の粒度分布 、 b 、 c Ru / SnO 2 ; d 、 e 、 f Ru / Sn 0.67 Ti 0.33 O 2 ; g 、 h 、 i Ru / Sn 0.5 Ti 0.5 O 2 ; j 、 k 、 l Ru / Sn 0.33 Ti 0.67 O 2 ;および m 、 n 、 o Ru / TiO 2
触媒の表面特性
基本状態と表面組成をさらに決定するために、XPS分析を実施しました。図8は、Sn x のSn3d、Ti 2p、O 1s、およびRu3dのXPSスペクトルを示しています。 Ti 1- x O 2 サポートとRu / Sn x Ti 1- x O 2 触媒。 Sn 3d 3/2 のXPS結合エネルギー値 およびSn3d 5/2 Sn 4+ の特徴である486.6–487.5 eVと494.9–496.1eVでそれぞれ観測されます。 Sn x の種 Ti 1- x O 2 サポートまたはRu / Sn x Ti 1- x O 2 触媒。興味深いことに、Sn 3d 3/2 の結合エネルギー およびSn3d 5/2 Sn 4+ の導入後、より高い値にシフトしました 、Sn 4+ の一部を示します Ti 4+ を交換してください サイトとTiO 2 との強い相互作用を持っています 、XRDと一致しています。また、酸素空孔は、より低い価数のSn δ+ によって作成される可能性があります。 [5]。 Ti 2p 3/2 に対応する2つのピーク およびTi2p 1/2 Ti 2pのXPSスペクトルで458.7–459.9 eVおよび464.3–465.8 eVで観察され、Ti 4+ およびTi 3+ サンプルに存在し、Ti 2p 3/2 の結合エネルギー値 およびTi2p 1/2 Sn 4+ の増加に伴い、より高い結合エネルギー値にシフトしました 、さらに酸素空孔の存在を証明します。表3から、XPSによるSn / Tiモル比が理論計算よりもわずかに高いことが観察され、Snが触媒の表面で濃縮され、より多くの酸素空孔が生じることがわかります。 Sn(1.96)の電気陰性度はTi(1.62)の電気陰性度よりも大きいため、つまり、Snの電子捕獲能力はTiの電子捕獲能力よりも強く、酸化還元平衡(Sn 4+ + Ti 3+ →Sn δ+ + Ti 4+ )右にシフト[32]。
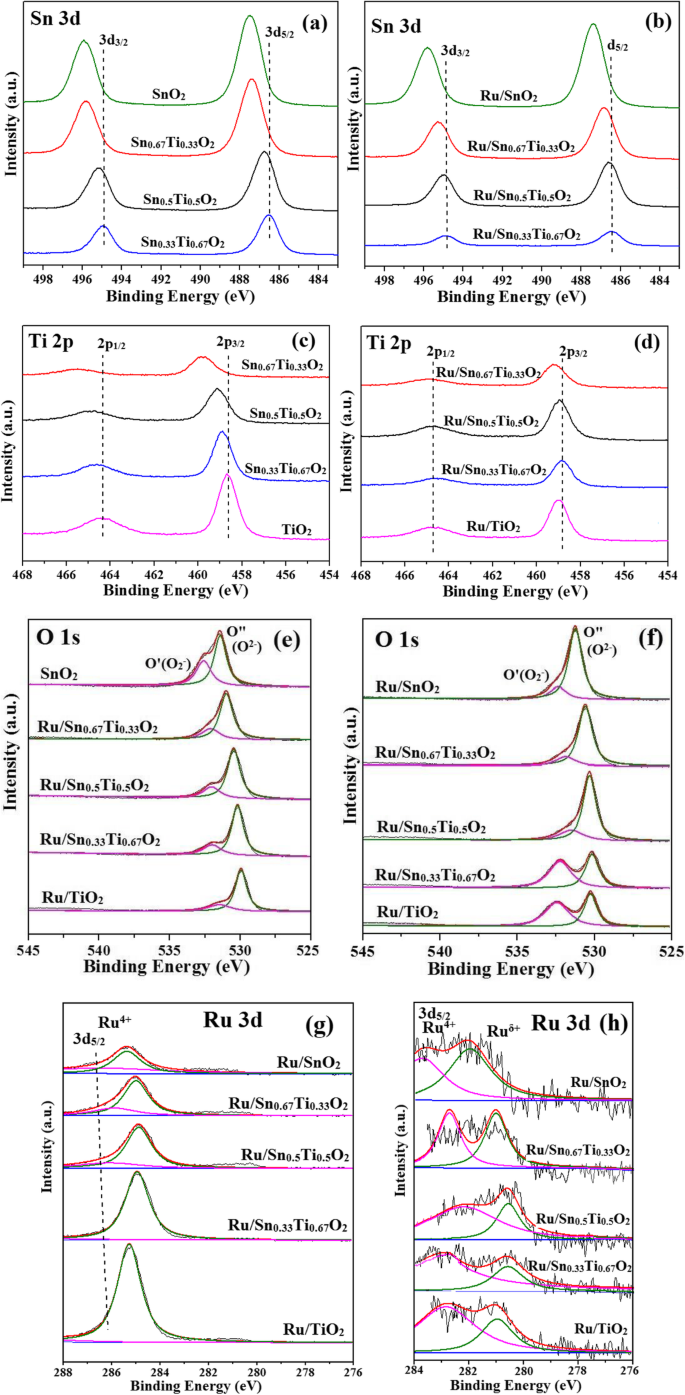
XPSスペクトル(Sn3d( a 、 b )、Ti2p( c 、 d )、O1s( e 、 f )およびRu3d( g 、 h ))Sn x の Ti 1- x O 2 サポートとRu / Sn x Ti 1- x O 2 触媒
O 1sイオン化機能の高分解能スペクトルは、ガウス機能と数値的に一致しており、2つのピークにデコンボリューションされています[5]。より高い結合エネルギー(O ’、532.1 eV)は、(O 2 を形成した化学吸着された酸素によって引き起こされます − 、O − 、またはO 2 2- ) 種族。 However, the O” (529.9 eV) is the characteristic peak of O 2− on the surface of metal oxides. Interestingly, the binding energy of O 1s shifted to higher values after the addition of Sn 4+ 。
Ru 3d spectra present Ru 4+ and lower value Ru δ+ 。 The signal of Ru 3d5/2 is often used to analyze the charge state of the Ru species, since another Ru 3d3/2 overlaps with C 1s at around 284.0 eV [40]. The binding energy of 282.0–283.5 eV is assigned to Ru 3d5/2 , which corresponded to Ru 4+ 。 The lower binding energy at around 280.2–281.7 eV is attributed to lower state Ru δ+ , and the Ru δ+ relative ratio in Ru/Sn0.67 Ti0.33 O 2 reaches 53.9%, which is higher than other catalysts. It could be explained that the strong interaction between Sn0.67 Ti0.33 O 2 and Ru caused a larger amount of surface reactive oxygen species [26].
XPS and EDS analyses are performed to determine the surface and bulk composition of the samples as shown in Table 2. Surface and bulk Ru analysis shows that Ru/Sn0.67 Ti0.33 O 2 has the highest surface Ru (0.69 wt.%) and bulk Ru (0.40 wt.%) among all the catalysts, indicating that the active component Ru is more evenly distributed on the Sn0.67 Ti0.33 O 2 support, and more Ru species enters the internal of Sn0.67 Ti0.33 O 2 to form a strong interaction.
<図> 図>In order to further investigate the reduction performance of the Ru/Snx Ti1−x O 2 catalysts, temperature-programmed reduction studies are performed (Fig. 9). The shapes of these H2 -TPR profiles are almost identical. The reduction peaks of Ru/Snx Ti1−x O 2 are divided into two parts:the low-temperature reduction peaks 80–270 °C are associated to the lower state Ru δ+ reduced from RuO2 and a significant amount of Sn 4+ which could be reduced to lower valent Sn δ+ or can be attributed to the reduction of surface oxygen [41], while the high-temperature reduction peaks 600–640 °C are associated to Sn 0 reduced from Sn δ+ or the reduction of bulk oxygen of catalysts [26, 42], which is consistent with XPS results. The reduction temperature of Ru/Snx Ti1−x O 2 moves towards lower temperature, peaks broaden and H2 consumption increase with the addition of Sn, and hydrogen consumption from the H2 -TPR measurements are shown in Table 3. The dispersion of active components on the surface of the samples has a significant effect on the reduction of surface oxygen, and hydrogen could be more easily activated with higher dispersion of Pd, resulting in the increase of H2 consumption [43]. Therefore, we can infer that the introduction of Sn significantly increased the dispersion of Ru on the carrier, which may have resulted from the formation of Snx Ti1−x O 2 solid solution. The results are in good agreement with XRD and TEM. Because the reduction of TiO2 is usually difficult to conduct at low temperature, there are no peaks of the TiO2 reduction observed during the H2 -TPR from 50 to 800 °C [15]. Nevertheless, the Ru/Sn0.67 Ti0.33 O 2 still exhibits a higher H2 consumption.
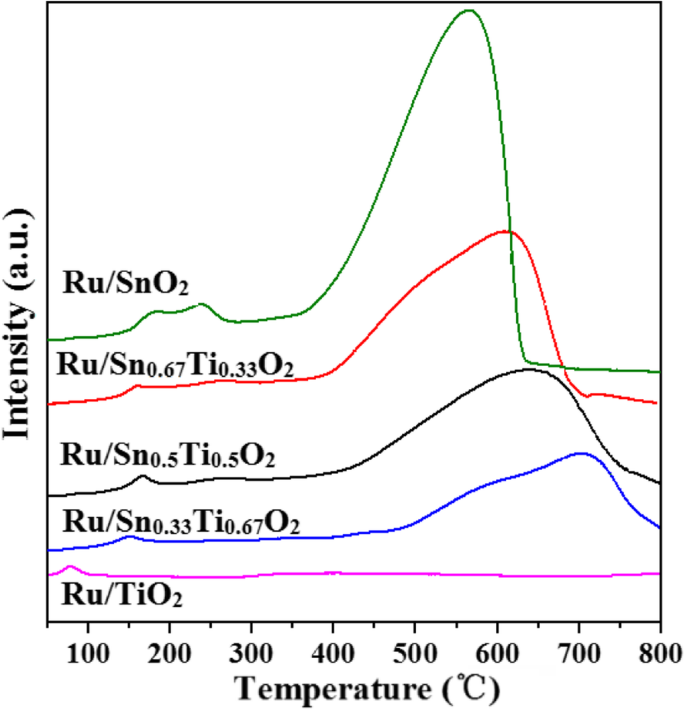
H 2 -TPR spectra of Ru/Snx Ti1−x O 2 catalysts
O 2 -TPD experiments (Fig. 10) of Ru/Snx Ti1−x O 2 samples are imposed to gain insight into the mobility of surface and lattice oxygen. The signal at low temperature (<200 °C) is attracted by the desorption of surface chemisorbed oxygen (O2 − 、O 2 2- , or O − species); the main peak centered at 280 °C or 500 °C which is attributed to the desorption of the structure oxygen species, and the peaks above 600 °C are assignable to the desorption of the lattice oxygen (O 2− ) species [44]. The incorporation of Sn increased the adsorbed oxygen species and shifted to a lower temperature [45]. The results indicate that the incorporation of Sn improved the oxygen activation ability of the Ru/Snx Ti1−x O 2 samples and the interaction between the carriers Snx Ti1−x O 2 and active component Ru [46, 47].
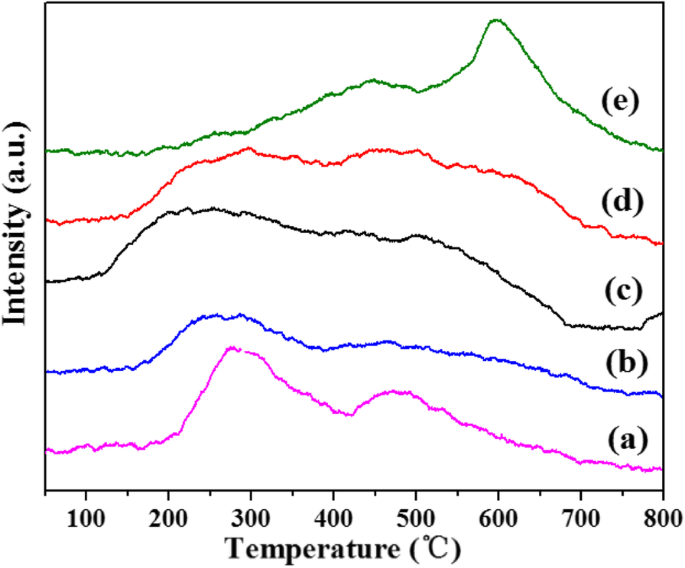
O 2 -TPD spectra of Ru/TiO2 ( a ), Ru/Sn0.33 Ti0.67 O 2 ( b ), Ru/Sn0.5 Ti0.5 O 2 ( c ), Ru/Sn0.67 Ti0.33 O 2 (d ), and Ru/SnO2 catalysis
CO and/or O2 Interaction with these Samples
The in situ FI-IR spectra of CO adsorption are recorded to further investigate the effect of the ruthenium oxide species, as shown in Fig. 11. The band located at 2052 cm −1 is attributed to linear CO adsorbed on reduced Ru crystallites (Ru δ+ –CO), the band at 2140 cm −1 and 2075 cm −1 can be assigned to two different types of multicarbonyl species on partially oxidized Ru sites (Ru n+ (CO)x ), and the band at 1765 cm −1 is attributed to (Snx Ti1−x O 2 )Ru–CO species [48, 49]. The Ru δ+ –CO adsorption peaks at room temperature indicate the presence of some lower state Ru δ+ species. This is in agreement with the XPS results. However, the desorption temperature of the Ru δ+ –CO peak is related to the Sn/Ti ratio and temperature. As the temperature increases, the peak intensity enhances firstly and then decreases gradually. Simultaneously, the CO adsorption peak moves to a higher wave number (2052 cm −1 at 25 °C and 2060 cm −1 at higher temperatures). This red-shift indicates that Sn 4+ has stronger electron-donating capability [50]. For the Ru/SnO2 , Ru/Sn0.5 Ti0.5 O 2 , Ru/Sn0.33 Ti0.67 O 2 , and Ru/TiO2 samples, the CO maximum adsorption peak on Ru δ+ appears at about 200 °C and disappears basically at 300 °C. For the Ru/Sn0.67 Ti0.33 O 2 sample, the CO maximum adsorption peak on Ru δ+ appears at about 200 °C, which can be observed clearly even at 300 °C. It can be concluded that Ru δ+ is much more stable in Ru/Sn0.67 Ti0.33 O 2 sample, which can provide more CO adsorption sites than in the other samples.
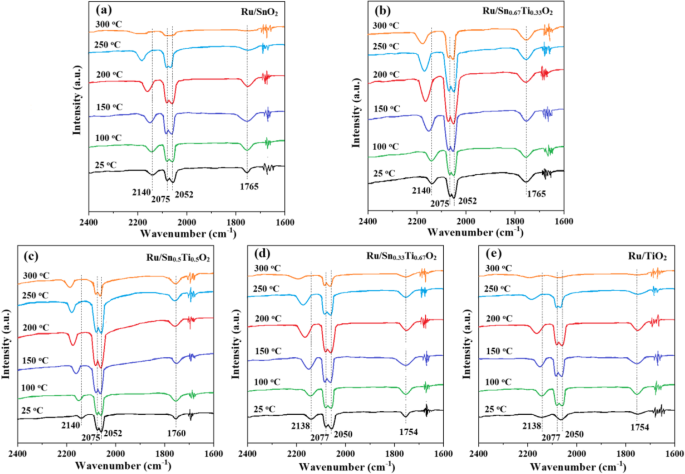
In situ FI-IR spectra of the 10% CO/Ar interaction with a Ru/SnO2 、 b Ru/Sn0.67 Ti0.33 O 2 、 c Ru/Sn0.5 Ti0.5 O 2 、 d Ru/Sn0.33 Ti0.67 O 2 、 e Ru/TiO2 at different temperatures
Possible Reaction Mechanism over the Ru/Snx Ti1−x O 2 Catalysts
According to the characterizations mentioned above, a possible reaction mechanism of CO and C3 H 8 oxidation is proposed and schematized in Fig. 12. Based on the XPS results, electrons migrate between Ru and Snx Ti1−x O 2 solid solution; because the electronegativity of Ru (2.22) is larger than that of Ti (1.62) and Sn (1.96), the electrons will transfer from the Snx Ti1−x O 2 solid solution to Ru 4+ , in which lower state Ru δ+ will be generated. Meanwhile, –Ti 4+ –O–Sn 4+ – species are oxidized and more oxygen will be absorbed on the surface of Snx Ti1−x O 2 solid solution, which can provide oxygen to the oxidation reaction of CO and C3 H 8 。 At the same time, the by-products produced in the oxidation process will also be adsorbed on the surface of Snx Ti1−x O 2 solid solution, which will not deteriorate the activity of Ru δ+ species. It is also the reason for the high stability of the catalysts. Moreover, the lower state Ru δ+ species have more metal properties, which play a crucial role in the activation of CO and C3 H 8 [40]. Compared with Ru/TiO2 and Ru/SnO2 , high dispersion of Ru on Snx Ti1−x O 2 solid solution is also an important cause for their excellent activity and stability. Based on O2 -TPD analysis, O2 is first adsorbed on the surface of catalysts to form O2 − species and CO and C3 H 8 adsorbed on Ru δ+ species react with O2 − species to produce CO2 and H2 O, which is a Langmuir-Hinshelwood mechanism.
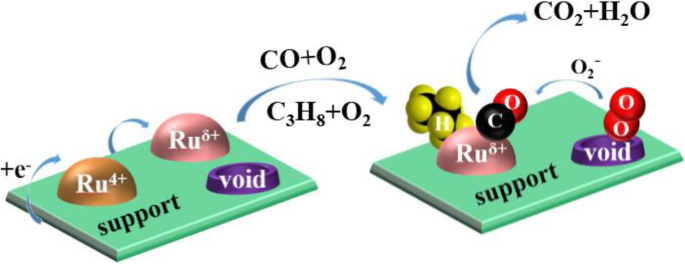
Possible reaction mechanism of CO and C3 H 8 over Ru/Snx Ti1−x O 2
Conclusions
A series of Ru/Snx Ti1−x O 2 catalysts were prepared by a one-step hydrothermal method for the catalytic oxidation of CO and C3 H 8 。 The preparation conditions of Ru/Snx Ti1−x O 2 catalysts were optimized for CO oxidation reaction. Ru/Sn0.67 Ti0.33 O 2 catalyst shows best CO catalytic activity and stability at low temperature under the condition of hydrothermal temperature at 180 °C, hydrothermal time at 24 h, and calcination temperature at 400 °C.
The effects of different molar ratios of Sn/Ti on the catalytic properties of Ru/Snx Ti1−x O 2 catalysts for CO and C3 H 8 were investigated under the optimum preparation conditions. The results show that the Ru/Sn0.67 Ti0.33 O 2 catalyst exhibits better low-temperature activity and stability. The conversion of CO reached 90% at 240 °C, and T 50 of which keeps at 180 °C. The complete conversion of C3 H 8 could be achieved at 500 °C, and its T 50 remains at 320 °C. The excellent catalytic activity of Ru/Sn0.67 Ti0.33 O 2 catalyst is attributed to the factors listed as follows.
- (1)
The successful incorporation of Sn 4+ into the TiO2 lattice to replace Ti 4+ forms a homogeneous solid solution (–Sn 4+ –O–Ti 4+ – species), which enhances the interaction between active component Ru and carrier Snx Ti1−x O 2 。 The crystal growth of the anatase phase can be inhibited by the introduction of Sn 4+ , which results in the presence of the rutile phase.
- (2)
Ultrafine Ru nanoparticles (~ 5 nm) are highly dispersed on Snx Ti1−x O 2 support, suggesting that the introduction of Sn 4+ could not only prevent grain agglomeration and induce a smaller grain size, but also produce more defects such as oxygen vacancies.
- (3)
CO and C3 H 8 species can be absorbed on Ru δ+ sites; O 2 − is formed by the adsorption of O2 on the oxygen vacancies. The adsorbed CO and C3 H 8 react with O2 − to produce CO2 and H2 O。
データと資料の可用性
All data generated or analyzed during this study are included in this published article and supporting information.
略語
- XRD:
-
X線回折
- ベット:
-
ブルナウアー-エメット-テラー
- FT-IR:
-
フーリエ変換赤外
- TEM:
-
透過型電子顕微鏡
- XPS:
-
X線光電子分光法
- H2 -TPR:
-
H 2 -temperature-programmed reduction
- O2 -TPD:
-
Temperature-programmed oxygen desorption
- DOC:
-
Diesel oxidation catalysts
- SCR:
-
Selective catalytic reduction
- DPF:
-
Diesel particulate filter
- SOF:
-
Soluble organic fraction
ナノマテリアル
- コバルトをドープしたFeMn2O4スピネルナノ粒子の調製と磁気特性
- TiO2ナノ流体に向けて—パート1:準備と特性
- SrTiO3修飾ルチルTiO2ナノファイバーのワンステップエレクトロスピニングルートとその光触媒特性
- Sb /パリゴルスキー石(PAL)ナノ粒子の調製と強化された接触水素化活性
- Li-Nb-O化合物の調製と光触媒性能に及ぼすLi / Nb比の影響
- Au @ TiO2卵黄シェルナノ構造の調製とメチレンブルーの分解および検出へのその応用
- 中空構造LiNb3O8光触媒の調製と光触媒性能
- PPy被覆MnO2ハイブリッドマイクロ材料の調製とリチウムイオン電池のアノードとしてのそれらの改善されたサイクル性能
- 光触媒性能が向上した新規Bi4Ti3O12 / Ag3PO4ヘテロ接合光触媒
- パラジウム(II)イオンインプリント高分子ナノスフェアの調製と水溶液からのパラジウム(II)の除去
- 1D混合二元酸化物CeO2-LaOx担持金触媒の合成とCO酸化活性